1. Introduction
With the rapid advancement of microfabrication and nanofabrication technologies, high-aspect-ratio (HAR) structures, characterized by heights or depths significantly exceeding their lateral dimensions, have garnered widespread attention[1,2]. The unique mechanical, optical, and chemical properties of these structures greatly enhance their application potential in various fields, including microelectronics[3], biomedicine[4], and optical devices[5]. The high surface area-to-volume ratio and inherent structural strength of HAR structures have facilitated innovative solutions to complex engineering[6,7]. However, in general, the production of HAR structures is complex, requiring precise control over materials, scale, and accuracy, often involving techniques, such as focused ion beam milling, deep etching, nanowire growth, and charged aerosol jet nanoprinting[8].
Recently, laser direct writing (LDW) technology has received widespread attention for its simplicity and cost-effectiveness[9,10]. It is extensively used to create vertically aligned polymers or micropillar arrays, providing a controlled three-dimensional environment suitable for applications, such as cell alignment[11,12], cell deformation[13], and the control of stem cell differentiation[14,15]. For example, Maibohm et al. utilized a low numerical aperture objective lens in parallel LDW processes to fabricate periodic three-dimensional microstructure arrays for cultivating living HeLa cells[16]. Additionally, Cadarso et al. employed two-photon polymerization and multiple exposure techniques to successfully produce ridges up to 9.8 µm high and 350 nm wide, achieving HAR structures with aspect ratios over 28 with vertically smooth sidewalls[17]. Further, Maibohm et al. designed and created a 3D lattice structure with niches of various sizes to study cell adhesion and migration, which also enhanced fluorescence imaging[18]. Further advancements include the nanopillar printing method[19], which enabled precise control over HAR structures, as well as studies on the height and width ratios of printed lines under various parameters[20], which have pushed the boundaries of LDW’s capabilities. Moreover, HAR structural applications like metalenses have shown great promise in fields such as optics[21]. These technologies have pushed the limits in terms of structural robustness, size, accuracy, and integration with existing technologies[22,23].
Although existing LDW technologies, such as two-photon polymerization and multiple exposures, are adept at creating structures with high aspect ratios and smooth sidewalls, there remains a notable gap in the development of smaller, narrower feature HAR nanowire structures. In this Letter, we introduce a method that leverages an LDW system to process HAR lines (HARLs) and structures. Utilizing a high numerical aperture objective lens and photoresist supplied by Nanoscribe, in conjunction with an 800 nm femtosecond laser for two-photon polymerization, our system facilitates the straightforward fabrication of microscale HAR structures. This method eliminates the need for complex optical shaping devices or repetitive operations. By finely tuning various laser processing parameters, we achieve precise control over the dimensions of the HAR structures, attaining minimum lateral widths of 37 nm, sidewall heights of 375 nm, and lengths of 450 nm, resulting in HARLs with an aspect ratio of 10. This result is comparable to that achieved with STED LDW[24,25] or suspended-line techniques[26], yet the fabrication process is significantly simpler. Additionally, we have successfully fabricated large-scale HAR three-dimensional structure arrays, proving the versatility of the potential of this technique across diverse applications in biomedical engineering, precision engineering, and optical meta-surfaces.
Sign up for Chinese Optics Letters TOC Get the latest issue of Advanced Photonics delivered right to you!Sign up now
2. Experimental Setup
The experimental setup, illustrated in Fig. 1(a), utilizes a femtosecond laser (Mai Tai, Spectral Physics) with a central wavelength of 800 nm, a pulse width of 100 fs, and a repetition rate of 80 MHz for two-photon polymerization to fabricate HAR structures. Laser power is finely controlled via a power control module consisting of a half-wave plate (HWP) and a polarizing beam splitter (PBS). A shutter (S) is used to control the required exposure time, and a Glan–Thompson prism (G) is employed to adjust the polarization state during processing. The laser is focused on the target plane using a high numerical aperture objective (OL, UPlanSApo , Olympus). A piezoelectric stage (P-733.3 XYZ, Physik Instrument) provides precise three-dimensional positioning, ensuring high-precision localization.
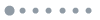
Figure 1.(a) Schematic diagram illustrating the optical path in the LDW system. L1, L2, lenses; HWP, half-wave plate; PBS, polarizing beam splitter; S, shutter; BS, beam splitter; G, Glan-Thompson prism; OL, objective lens. (b) Schematic diagram of photoresist placement. (c) Schematic diagram of the HARL processing method.
As shown in Fig. 1(b), the method involves encapsulating the required photoresist between two cover glass substrates, where the photoresist thickness is approximately 2 µm. The lower glass substrate acts to separate the immersion oil from the photoresist, whereas the upper glass substrate provides a stable processing platform. The process is started by rapidly exposing the photoresist at a designated distance to form two pillars with height at both endpoints. Subsequently, a wire is drawn from one pillar to the opposite pillar at a fixed exposure speed, ultimately achieving the HAR structure, as shown in Fig. 1(c). The negative photoresists employed in the experiments, primarily IP-L780 and IP-Dip (provided by Nanoscribe), feature excellent biocompatibility and non-toxicity, making them suitable for biomedical applications. These photoresists offer sub-micron resolution in structure fabrication and exhibit a low shrinkage rate, which enables higher aspect ratios. Additionally, incorporating 0.5% (mass fraction) DETC (7-diethylamino-3-thenoylcoumarin) into the photoresist enhances sensitivity, reducing the power needed for processing and accelerating the manufacturing process.
After fabrication, the sample is sequentially soaked in acetone for 1 min and washed in ethanol for 3 min to ensure thorough cleaning. Subsequently, a 90 s sputter coating is applied to enhance conductivity and improve image contrast for the scanning electron microscope (SEM) imaging. Subsequent imaging is performed using an SEM, with the samples tilted at 54° under the SEM observation to obtain a clear view of the lateral profiles.
3. Experimental Results
To achieve precise control of the inter-pillar distance, the LDW power is adjusted to 11 mW by rotating the HWP, and the polarizer G is tuned to ensure alignment of the laser’s polarization direction with the movement direction of the piezoelectric stage. The exposure time for the pillars is maintained at 500 ms, with processing conducted at a direct writing speed of 200 µm/s for HARL fabrication. During processing, the inter-pillar distance is incrementally adjusted, starting from an initial distance of 500 nm and gradually increasing to a final distance of 3.5 µm.
Figure 2(a) illustrates the top and side views of the HARLs obtained using the photoresist IP-L780. Observations indicate that when the inter-pillar distance is narrow, adhesion between pillars prevents the formation of the anticipated HARL sidewalls. However, at an inter-pillar distance of 1 µm, the structure reached its minimum lateral width and the highest sidewall height, exhibiting the maximum aspect ratio. With further increases in inter-pillar distance, the lateral dimensions gradually expand, while the aspect ratio correspondingly decreases. When the inter-pillar distance becomes too wide to support the sidewall structures, collapse of the structure occurs. Therefore, subsequent discussions primarily focus on the impact of various parameters at an inter-pillar distance of 1 µm. Figure 2(b) illustrates the direct writing results obtained using another photoresist, IP-Dip. Its processing characteristics are similar to those of IP-L780, indicating the suitability of this LDW technique for various photoresist materials.
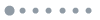
Figure 2.Experimental results of the fabricated HARLs with different lengths. (a) IP-L780 photoresist. (b) IP-Dip photoresist. The top and side views of the HARLs are shown in the top and bottom halves of (a) and (b), respectively.
To further understand the impact of the pillar height on the HARLs, the writing laser power is fixed at 11 mW, with an inter-pillar distance of 1 µm, and the polarization is aligned parallel to the processing direction. The exposure time for the pillar fabrication are controlled at 500, 700, and 900 ms, respectively. Additionally, during the exposure of the pillars, the piezoelectric stage is moved vertically downward at a speed of 5 µm/s to increase the distance from the upper glass substrate. The processing speed of the HARLs remained constant at 200 µm/s. The results are illustrated in Fig. 3.
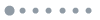
Figure 3.Experimental results of the fabricated HARLs with different pillar processing time. (a) 500 ms, (b) 700 ms, and (c) 900 ms.
As shown in Fig. 3, increasing the exposure time and the movement of the stage leads to a corresponding increase in pillar height. When the pillar height reaches 1.9 µm, the lateral width of the HARLs is 64 nm, with a sidewall height of approximately 550 nm, resulting in an aspect ratio of 9:1. As the pillar height increases, the lateral width gradually increases, while the aspect ratio remains relatively constant. Although lower pillar heights do not enhance the aspect ratio advantage of the HARLs, they result in longer lines. When the pillar height is 1.1 µm, the length of the HARLs reaches 450 nm. This occurs because prolonged exposure increases the pillar width, thereby reducing the space available for the sidewalls. Consequently, lower pillar heights may offer certain benefits for fabricating subsequent complex HAR structures.
Next, we investigate the impact of LDW speed on the geometric dimensions of the HARLs. To do this, we keep the direct writing power and polarization constant and use previously determined parameters for pillar height and inter-pillar spacing.
As shown in Fig. 4, the SEM images reveal the relationship among the processing speed, the lateral width, and the sidewall height of the HARLs. With an increase in processing speed, the lateral dimensions of the HARLs continuously decrease and their height declines, but the aspect ratio gradually increases. At a direct writing speed of 500 µm/s, the cross-sectional size reduces to 33 nm. However, Fig. 4(c) indicates that excessive processing speed causes breakage at the base of the HARLs, compromising their structural integrity. This defect arises from the insufficient adhesion between the photoresist and the glass substrate coupled with insufficient cumulative exposure to reach the photopolymerization threshold under high-speed processing conditions. Additional experiments revealed that a direct writing speed of 400 µm/s resulted in the fabrication of complete vertical HARL structures. These structures exhibited lateral feature dimensions of approximately 37 nm and achieved an aspect ratio of 10:1. This indicates that this type of high-aspect-ratio laser system and methodology can easily produce structures with feature sizes beyond the diffraction limit, demonstrating the significant potential for precision nanomanufacturing.
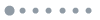
Figure 4.Experimental results of the fabricated HARLs with different direct writing speeds. (a) 200 µm/s, (b) 400 µm/s, and (c) 500 µm/s.
We also analyze the impact of the direct writing power and polarization on HARLs. Compared to Fig. 4(b), we rotated the HWP to increase the writing laser power from 11 to 16 mW, with other parameters unchanged. As depicted in Fig. 5(a), the results demonstrate that with the increase in laser power, the lateral dimensions and shape of the microstructures change accordingly, leading to a gradual decrease in the aspect ratio of the HARLs relative to their lateral dimensions. Therefore, selecting appropriate exposure parameters is crucial in achieving the desired aspect ratio during the fabrication of the HARLs.
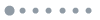
Figure 5.(a) Experimental results of the fabricated HARLs with high LDW power (16 mW). (b) Experimental results of the fabricated HARLs with 90° polarization states.
Additionally, this study investigates the impact of different polarization states on the HARLs. By adjusting the polarization (G), the angle between the polarization direction and the machining axis changes from 0 [Fig. 4(b)] to 90 deg [Fig. 5(b)]. Experimental results, as depicted in Fig. 5(b), indicate that the polarization state has a relatively limited impact on the dimensions of the microstructures. This finding indicates that polarization factors may not require special attention during microstructure fabrication, providing a basis for simplifying the fabrication workflow.
To further verify the practicality of this HARL fabrication technology, we conducted a series of fabrication experiments. The HAR microstructures are created with specific parameters: processing speed is set at 100 µm/s, the laser power is set at 10 mW, and the pillar exposure time is 500 ms. The resulting structure, illustrated in Fig. 6, is observed from multiple angles to assess this high aspect ratio. Experimental results indicated that the resultant microstructures possessed a lateral size of approximately 90 nm and a sidewall height of 780 nm, achieving an aspect ratio reaching 10:1. Such size-adjustable grids generated in the processing offer additional possibilities for sub-micron 4D printing[27]. Furthermore, the achieved aspect ratio demonstrates that this processing technique can effectively fabricate microstructures with complex high aspect ratios. HAR structures hold great promise in the biomedical field. HAR microstructures, with their larger surface area, can be used in highly sensitive biosensors. Additionally, in the field of microfluidics, HAR structures can significantly optimize fluid flow characteristics in microchannels and improve mixing efficiency. This HAR structure is useful in generating periodic structures to produce structural color pattern arrays[28].
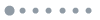
Figure 6.Experimental results of the fabricated HAR array microstructure. (a) The isolated HAR cell microstructure. (b) The array of the HAR microstructures. (c) The top view of the HAR array microstructure. (d) The side view of the HAR array microstructure.
4. Discussions and Conclusions
In this study, we present a method for achieving HAR nanostructures using LDW technology and systematically investigate the effect of various controllable parameters on the fabrication process. By optimizing these parameters, we successfully fabricated HAR structures with a lateral width of 37 nm and an aspect ratio of 10:1. Furthermore, the effectiveness of the system and method is experimentally verified through the fabrication of three-dimensional arrays with high aspect ratios, demonstrating the controllability of aspect ratio and lateral width.
The formation of HARLs may be influenced by the surface tension of the photoresist[29,30]. Reducing surface tension during post-development is crucial for maintaining the integrity of the structures. This requirement differs from the formation mechanism of suspended lines in LDW. Achieving high-quality HARL microstructures requires careful balancing of the processing speed and power to ensure proper adhesion between the photo-cured material and the substrate, preventing distortion or defects. Moreover, using radially polarized light with a longer focal length can provide more concentrated and deeper energy distribution[31], facilitating the formation of HAR structures[32]. Additionally, employing a thicker layer of photoresist or developing photoresists with a higher Young’s modulus also contributes to increasing the aspect ratio of the structures. A thicker photoresist layer provides more processing depth, which further enhances the formation of high-aspect-ratio structures.
In summary, this study not only systematically validates the impact of various parameters on the aspect ratio of HARLs but also provides an effective technological path for manufacturing complex microstructures. It also offers experimental support for further optimization of high aspect ratio microstructures. This method demonstrates significant potential and scalability in applications requiring precise spatial control, such as micro-optical components, biomedical applications, sensors, and microfluidic devices.