1. Introduction
Multiphoton fluorescence microscopy has the advantages of intrinsic optical sectioning, reduced out-of-focus photobleaching, and increased imaging depth, and it has become an indispensable imaging tool for biophotonics[1–4]. Especially in the field of neuroscience, multiphoton fluorescence microscopy can be leveraged to record the in vivo neuronal populations and activities under physiological conditions[5–13], facilitating the structural and functional study of brain science.
Multiphoton-excited fluorescence through multiphoton absorption exhibits small excitation cross-sections[14,15], resulting in weak fluorescence signals as the nature of multiphoton fluorescence microscopy. One straightforward solution for enhancing the fluorescence signal is to simply increase the power of the excitation pulses. However, increasing excitation power gives rise to a higher risk of photoinduced damage to the biological samples and both photobleaching and photodamage must be considered[16]. As a result, a balanced solution of obtaining high fluorescence yield and keeping the fluorophores from photoinduced damage is highly desired. To this end, the strategy of using femtosecond pulses at high repetition rates of several hundred MHz to GHz as the excitation source has been proposed for nonlinear imaging[17]. High-repetition-rate femtosecond pulse lasers generated by various methods, like splitter-based pulse splitting[17] and high-repetition-rate fundamental mode-locking[18,19], have been applied to demonstrate the enhancement of fluorescence signals and the suppression of photoinduced damage in multiphoton fluorescence microscopy platforms. It is also worth noting that denser excitation pulses may lead to the population of the long-lived triplet state[20,21], such that a higher excitation frequency results in severe photobleaching. Moreover, with constant average power, the denser excitation pulses reduce their peak power, to some extent, preventing the deep tissue multiphoton imaging of scattering biological samples that require high peak power to increase imaging depth. An improved excitation solution of multiphoton excitation using high-repetition-rate femtosecond pulses is yet to be demonstrated for nonlinear imaging.
In this Letter, we introduce a new multiphoton excitation scheme using high-repetition-rate femtosecond pulses, called pixel-excitation gating, for high-sensitivity nonlinear imaging. The pixel-excitation gating is implemented in a customized galvo-based laser scanning two-photon excitation (TPE) microscope using a femtosecond pulse fiber laser at a repetition rate as the excitation source. An acousto-optic modulator (AOM) is used for gating the excitation femtosecond pulse train. For precisely gating within the pixel dwell time of the corresponding scanning point, the line trigger of the raster scanning scheme is synchronized with the driving signal of the AOM. We demonstrate the effectiveness of pixel-excitation gating for increasing the fluorescence signal of TPE imaging of different samples, including fluorescent microspheres, prepared mouse kidney slides, and tdTomato-labeled fixed mouse brain slices. In contrast to a continuous GHz pulse train, multiphoton excitation using pixel-excitation gating with a 25% duty cycle of the 10 µs pixel dwell time can enhance the fluorescence signal by up to -fold at constant average power.
Sign up for Chinese Optics Letters TOC Get the latest issue of Advanced Photonics delivered right to you!Sign up now
2. Results
2.1. Experimental setup
The setup of the TPE imaging system with pixel-excitation gating is schematically depicted in Fig. 1. The TPE source is a home-built GHz femtosecond pulse Yb-doped fiber laser, and its details can be found in our previous work[22]. This fiber laser can provide watt-level ultrashort pulses at a repetition rate with a central wavelength of 1055 nm and a pulse duration of 220 fs. To implement the pixel-excitation gating, the pulse train is launched into a free-space AOM (IntraAction, AOM-402AF3). The driven pattern of the AOM is generated by a triggered arbitrary waveform generator (AWG, Rigol, DG 4202). The line trigger of the raster scanning is synchronized to the AWG signal, i.e., the illumination and scanning start at the same time. The line trigger is provided by a data acquisition (DAQ) card (National Instruments, PCIe-6321). A square waveform with variable duty cycles is used for the pixel-excitation-gating. The period of the square waveform is set to 10 µs, equal to the pixel dwell time. The duty cycle of the square waveform can vary between 20% and 100%. The gated pulses are sent to a galvo-based X–Y scanner for laser beam scanning. The driven signal of the galvo is provided by another DAQ card (National Instruments, PCI-6110). The beam size to the microscope is manipulated by the combination of two Keplerian telescopes that have a reduction ratio of and expansion ratio of , i.e., and , respectively. The beam size can fully fill the back aperture of the microscope objective (Olympus, XLPLN25XSVMP2). The backscattered fluorescence emission is collected by the same objective and then filtered out by a 775 nm cutoff dichroic mirror before being projected onto the photomultiplier tube (PMT). The analog output of the PMT is digitized by the DAQ card, which generates the galvo-driven signal as well.
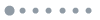
Figure 1.The schematic diagram of the pixel-excitation-gating enhanced GHz femtosecond pulse TPE microscope. The pixel-excitation-gating excitation is implemented by modulating the excitation GHz pulse train with an acousto-optic modulator (AOM), which is driven by a modulation waveform signal synchronized to the galvo-based raster scanning. The right bottom inset shows the temporal characteristics of the driven signals for the X-galvo, arbitrary waveform generator (AWG), and AOM. DAQ, data acquisition; PMT, photomultiplier tube; L, lens; Obj, microscope objective; DM, dichroic mirror.
2.2. TPE imaging of different samples
In this scenario, the optical beam precisely fires the gated pulse burst within the pixel dwell time of the corresponding scanning point, without losing the optical power that generates background noise. Thus, with similar optical power, the fluorescence signal intensity can be increased. The point spread function (PSF) of the TPE imaging system is measured using fluorescent microspheres with a diameter of 200 nm. The microspheres are imaged with 512 pixel × 512 pixel (corresponding to an 89 µm × 89 µm area), 10 µs pixel dwell time, and step size. A total of 50 Z-stack frames are averaged together to get the XY image of the PSF. The TPE image with a single microsphere is shown in the inset of Fig. 2(a). The measured full width at half-maximum (FWHM) of the PSF is 620 and on the X- and Y-axis directions, respectively.
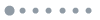
Figure 2.(a) The lateral resolution of the TPE microscope, measured using 200 nm fluorescent microspheres, under GHz femtosecond pulse TPE. The inset shows the image of a single 200 nm microsphere, averaged from 50 Z-stack frames. TPE images of 3 µm red fluorescent microspheres, excited by (b) GHz femtosecond pulses without pixel-excitation-gating, (c) with pixel-excitation-gating of 50% duty cycle and (d) 25% duty cycle. In the three cases, the same average power of 40 mW is applied. “R” indicates the raw image, while “G” indicates the image processed with a 2-pixel-radius Gaussian filter.
Then, the comparison of the fluorescence intensity excited without and with pixel-excitation gating is conducted using 3 µm fluorescent microspheres. The duty cycle of the pixel-excitation-gating modulation is set to either 50% or 25%. The pixel dwell time and average power are 10 µs and 40 mW, respectively, for all cases. The gain of the PMT set to a proper value cannot only avoid pixel clipping for the case using a 25% duty cycle, but it can also make the microspheres observable without pixel-excitation gating. The representative TPE images of the microspheres are shown in Figs. 2(b)–2(d). To remove Gaussian noise, 2-pixel-radius Gaussian filtering is applied to the raw images (indicated by “R”). The processed images are shown on the top right corners of Figs. 2(b)–2(d) (indicated by “G”). In contrast to the case without pixel-excitation gating, the fluorescence intensity is significantly enhanced with the pixel-excitation-gating illumination. The averaged fluorescence intensity of each selected microsphere (indicated with arrows) in the denoised image is calculated to quantify this fluorescence intensity improvement. To eliminate the influence of the translations in both the X and Y axes on the comparison, intensity-based image registration is utilized to align the selected areas, and the denoised image captured using a 25% pixel-excitation gating duty cycle is used as the registration reference. The signal enhancements are - and 3.8-fold for the 50% and 25% pixel-excitation gating duty cycles, respectively. As expected, the enhancement of fluorescence intensity with pixel-excitation gating illumination has a nearly linear dependence on the inverse of the pixel-excitation gating duty cycle. When gating the excitation pulse train using a duty cycle under identical average power, it follows a -fold signal enhancement, while a excitation duration reduces the enhancement to -fold.
To assess the effect of the pixel-excitation gating on nonlinear imaging of real biological samples, a stained tissue slide (Invitrogen, FluoCells F24630) is utilized to perform TPE fluorescence imaging. This sample contains a 16 µm cryostat section of mouse kidney, labeled with DAPI (nuclei), Alexa Fluor 488 (glomeruli and convoluted tubules), and Alexa Fluor 568 (F-actin). The average power of the excitation femtosecond pulses keeps 30 mW. Figures 3(a) and 3(b) show TPE images of the convoluted tubules in the kidney section, under the illuminations without pixel-excitation-gating and with pixel-excitation-gating of 25% duty cycle, of the 10 µs pixel dwell time, respectively. The pixel dwell time is the same for the two cases, i.e., 10 µs. With pixel-excitation-gating, higher fluorescence yields can be obtained, which makes the labeled structure of the convoluted tubules clearly observed. The line-plot signal crossing a convoluted tubule after the 2-pixel-radius Gaussian filtering is employed to quantify the imaging improvement, as shown in Figs. 3(c) and 3(d), respectively. The results suggest that a -fold signal intensity enhancement can be obtained under pixel-excitation-gating illumination. Moreover, a noise estimation method[23] is applied to the raw images captured under these two illumination conditions and estimates the Poisson–Gaussian noise parameters, in which the Poisson noise is parameterized by and Gaussian noise is parameterized by , respectively. Without pixel-excitation-gating, the estimated and are and , respectively. Positive values of and imply that the TPE image has mixed Poisson–Gaussian noise. More specifically, a comparably large with a small means that high Poisson noise is present in the raw image. In other words, the noise of the raw image is dominated by the Poisson noise. With pixel-excitation-gating, the estimated and are and , respectively. Again, the positive values mean that the TPE image has a mixed Poisson–Gaussian noise, while the Gaussian noise is the main noise source, different from the case without pixel-excitation-gating. The different levels of peak power in these two cases may cause a change in the dominant noise source. In the case without pixel-excitation-gating, the continuous GHz pulse train leads to a peak power reduction, equivalent to a “low-light” illumination condition, in which the signal fluctuation is dominated by the shot noise, or the Poisson noise, due to the discrete nature of photons[24]. In the case of pixel-excitation-gating, a higher peak power results in the increase of fluorescence photon numbers[25], which makes the inherent Poisson distribution of the collected fluorescence photons tend toward a Gaussian distribution, namely a larger .
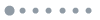
Figure 3.TPE imaging of the mouse kidney section with a region size of 245 µm × 245 µm, captured (a) without and (b) with pixel-excitation-gating of 25% duty cycle. All the images were captured at a 30 mW average power. (c) and (d) Line plots of the image along the convoluted tubule, as indicated by the yellow lines in (a) and (b), respectively.
Furthermore, the pixel-excitation-gating illumination is applied to image tdTomato-labeled neurons in a fixed mouse brain slice to assess the TPE imaging quality improvement. The average power of the excitation femtosecond pulses is fixed to 40 mW. The representative images are shown in Figs. 4(a) and 4(b). In addition to the raw images (indicated by “R”), 50 raw images are averaged together to obtain a clean image, indicated by “S.” In this case, the image denoising is implemented by image averaging, instead of Gaussian filtering, which can suppress the influence of background noise while keeping a sharp edge of the captured image, which might be beneficial for observing finer dendritic structures. Without pixel-excitation-gating, the fluorescence signal is relatively weak, and only the neuron body, namely soma, can be observed while the dendritic structure is buried in the background noise. By applying pixel-excitation-gating of a 25% duty cycle, a brighter fluorescence image is captured, and the soma, as well as the dendritic structure of the neuron, is significantly enhanced. Figure 4(c) shows the line plots along the soma and dendrite, indicated by “L1” and “L2,” respectively. For the soma, a -fold signal enhancement is obtained with pixel-excitation-gating. For the dendrite, a -fold signal intensity enhancement is achieved with pixel-excitation-gating.
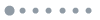
Figure 4.TPE imaging of neurons in a mouse brain slice with a region size of 327 µm × 327 µm, captured (a) without and (b) with pixel-excitation-gating of 25% duty cycle. All the images were captured at a 40 mW average power. “R” denotes the raw images, while “S” denotes the image averaged from 50 raw images. (c) Line plots of the images along the soma (indicated by L1) and dendrites (indicated by L2), as shown in (a) and (b). The solid and dashed lines indicate the cases with and without pixel-excitation-gating, respectively.
3. Conclusion
In conclusion, we have demonstrated a pixel-excitation gating TPE imaging using femtosecond pulses as the excitation source. The fluorescent microspheres, stained mouse kidney section, and labeled fixed mouse brain slice were employed to validate this new method under a constant 10 µs pixel dwell time. With pixel-excitation-gating of a 25% duty cycle, it can improve the TPE signal intensity by up to -fold. The application of pixel-excitation gating can resolve finer structures. The use of GHz femtosecond pulses enables more flexible temporal manipulation of the optical excitation, as the pulse train is dense. The pixel-excitation gating TPE using GHz femtosecond pulses can serve as an add-on unit to upgrade existing optical microscopes, and it is anticipated that the TPE signal enhancement of the pixel-excitation gating that leverages the GHz femtosecond pulse laser can open new opportunities for nonlinear imaging in applications requiring high sensitivity. Particularly, for living cell imaging, the application of GHz femtosecond pulses enables hours-long live-cell imaging of macrophage behavior with a reduced higher-order photobleaching effect[26], which makes the functional TPE observation of living samples possible.