Introduction
Infrared imaging technology plays a pivotal role across a diverse range of applications,spanning both defense and public sectors. This technology is crucial in areas such as medical diagnostics,nocturnal surveillance,security operations,environmental observation,pollutant detection,and molecular characterization[1-4]. Traditionally,the development of infrared detectors has been dominated by epitaxial materials such as Mercury Cadmium Telluride[5] and Indium Gallium Arsenide[6],as well as Indium Antimonide[7]. These materials are highly valued for their excellent sensitivity and performance across different infrared wavelengths. However,the complex manufacturing processes,high costs associated with epitaxial growth,and the need for cryogenic cooling systems to achieve optimal performance have focused their application range on military and industrial[8]. Over the last ten years,CQDs have seen remarkable advancements in the field of photodetection[9]. The appeal of CQDs lies in their size-tunable optical properties,which span the entire infrared spectrum,their cost-effectiveness in production,and their compatibility with direct application onto silicon readout integrated circuits(ROIC) for imaging purposes[10-13]. Consequently,CQDs hold the promise of significantly lowering the overall cost of sensors to nearly that of the silicon substrates they are applied to,while also introducing versatile new approaches to detector manufacturing.
However,current photodetectors based on HgTe CQDs,such as homojunction[14],photoconductive[15],trap-state photoconductive[11],planar p-n junction[16],and diode types[17],tend to exhibit high dark current. Planar p-n junctions in two-dimensional materials are commonly fabricated through the regulation by ferroelectric polarization fields[18-20]. These issues may arise from several factors: the spatial charge region of planar p-n junctions occupies a low proportion,the mismatch in barrier layer materials for diodes,and the insufficient density of the quantum dot films. Therefore,to develop a vertically stacked device structure with low dark current for integration with ROIC,it is crucial to address the selection of barrier layers,the quality of quantum dot synthesis,and the fabrication of high-quality thin films.
In this work,leveraging previously reported high-quality quantum dot synthesis and superlattice-like thin film fabrication techniques[21],and identifying ZnO QDs as a barrier layer,we have fabricated a HgTe/ZnO type-II heterojunction photodetector. Heterojunctions,compared to homojunctions,possess more abundant band information,allowing for the design of higher barriers,thereby more effectively suppressing dark current. This device demonstrates high rectification ratios and low dark currents at both room and low temperatures,presenting significant potential for future integration with ROIC.
1 Results and discussions
Figure 1(a) presents a detailed schematic of the device architecture,illustrating the HgTe CQDs/ZnO QDs heterostructure. The fabrication process involved the precise patterning of Ti/Au(5 nm/10 nm) metal electrodes using ultraviolet lithography,followed by deposition through electron beam evaporation. Subsequently,ZnO QDs were carefully deposited onto the pre-patterned electrodes and spin-coated at 2000 rpm for 30 seconds. The resulting film underwent annealing at 200 ℃ on a heating plate in ambient conditions for 1 hour. Next,the HgTe CQDs film was uniformly blade-coated onto the ZnO film and then subjected to heating at 45 ℃ for 5 minutes. Finally,a 70 nm indium tin oxide film was precisely deposited using dual ion beam sputtering to serve as the top transparent electrodes. In Figure 1(b) and 1(c),atomic force microscope(AFM) images provide a detailed view of the surface morphology of the HgTe and ZnO films,respectively. The HgTe film exhibits a surface roughness of 2.69 nm,indicative of a relatively smooth and compact surface. Similarly,the ZnO film displays a surface roughness of 577 pm,suggesting a uniform and flat surface conducive to forming a high-quality interface between the two materials. Figure 1(d) and 1(e) showcase the absorption spectra of HgTe and ZnO,respectively. The observed cutoff wavelengths are 3600 nm for HgTe and 370 nm for ZnO,indicating their respective absorption characteristics. This suggests that the fabricated heterojunction device is capable of responding to infrared radiation. Figure 1(f) provides a comprehensive X-ray photoelectron spectroscopy(XPS) analysis of ZnO nanostructures. The O 1s peak observed in ZnO QD can be decomposed into two distinct subpeaks. The subpeak at a higher binding energy(approximately 531.48 eV) is associated with absorbed water(O-H),while the subpeak at a lower binding energy(approximately 530.06 eV) is indicative of ZnO(O-Zn). Additionally,the peaks corresponding to Zn 2p and Zn 2pare found at 1 044.40 eV and 1 021.32 eV,respectively.

Table 1. Dark current level comparison based on different HgTe CQDs device structures
Table 1. Dark current level comparison based on different HgTe CQDs device structures
Device | T(K) | Dark current(A cm-2) | Ref |
---|
HgTe CQDs homojunction | 80 | 5 × 10-6 | [14] | HgTe CQDs planer p-n junction | 300 | 1 × 10-7 | [16] | HgTe CQD/SnO2 diode | 300 | 1 × 10-6 | [27] | HgTe CQD/ZnO heterojunction | 80/300 | 5.23 × 10-9/9 × 10-8 | This work |
|
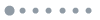
Figure 1.Basic physical characteristics of materials.(a) Device structure diagram of HgTe/ZnO heterojunction;(b) the AFM image of HgTe film;(c) the AFM image of ZnO film;(d)the absorption spectrum of HgTe film;(e) the absorption spectrum of ZnO film;(f) XPS spectra of Zn 2p and O 1s orbitals of ZnO thin films
For energy level characterization,Figure 2 presents the ultraviolet photoelectron spectroscopy of the ZnO film and HgTe film. The work function(WF) values of ZnO and HgTe are determined as 6.33 eV and 3.64 eV,respectively,using the equation ,where hv = 21.2 eV represents the photon energy of He Ⅰ light. The cutoff energy(Ecut) values for ZnO and HgTe are measured as 14.87 eV and 17.56 eV,respectively,as depicted in Figure 2(a) and 2(c). Furthermore,the Fermi levels of ZnO and HgTe are observed to be positioned 0.82 eV and 0.17 eV higher than the valence bands,as illustrated in Figure 2(b) and 2(d). Additionally,based on the bandgap values calculated from the absorption spectrum,the conduction band(EC) values are estimated to be 3.8 eV and 3.47 eV for ZnO and HgTe,respectively.
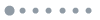
Figure 2.The UPS of HgTe CQDs and ZnO QDs:(a)-(b) Valence band spectrum and second electron cutoffs of ZnO;(c)-(d) Valence band spectrum and second electron cutoffs of HgTe
In accordance with the band information of each material,we depicted a schematic diagram of the band alignment for the heterojunction device,fabricating a type-II heterojunction as shown in Figure 3(a). When HgTe CQDs absorb incident light to produce excitons,under the separation by the built-in electric field,electrons flow from HgTe to ZnO and are collected through the gold electrode,while holes flow from ZnO to HgTe and are collected through the ITO. The optical photograph of our detector is displayed in Figure 3(b),with the effective working area of the device being 100 μm × 100 μm. To investigate the interface conditions of our fabricated device,we conducted scanning electron microscopy(SEM) imaging of the device's cross-section,obtaining a clear image of the cross-section,as shown in Figure 3(c),the interfaces between each layer are clearly visible. The clean interface of the heterojunction device,prepared with overlapping smooth films,provides potential for effective optoelectronic detection.
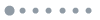
Figure 3.The HgTe CQDs/ZnO QDs heterojunction:(a) Energy band diagram of the device;(b) the optical image of device;(c) the Cross-sectional SEM image of device
To thoroughly assess the performance of the HgTe/ZnO heterostructure device,the figures of merit were carefully measured. We first tested the device's photodetection capability at room temperature,as shown in Figure 4. Figure 4(a) shows the current density-voltage(J-V) curves of the device with power density varying from 0 to 9 W cm-2 under a 520 nm laser illumination. The device demonstrated a high rectification ratio of near five orders of magnitude at ±0.75 V in the dark state,suggesting minimal series resistance and effective charge extraction[22]. Under reverse bias,the photocurrent closely follows a smooth curve,indicating that the photogenerated carriers are effectively collected under the influence of an external electric field. This smooth curve and high rectification ratio are crucial in practical applications,as any abnormal voltage fluctuations near the operating bias from the ROIC would cause negligible interference to the signal intensity,thereby enabling real imaging. Figure 4b illustrates the relationship between photocurrent and incident light power under self-driven conditions,where they follow a power-law relationship given by ,α calculated to be 0.98 through exponential fitting. The near-ideal value of α suggests that defects in the device minimally affect the generation and recombination of photogenerated electron-hole pairs,attributed to the near-perfect interfaces in our fabricated device[23]. Another important parameter is the linear dynamic range(LDR),representing the stability of the photodetector's response rate under varying light intensities. The formula for calculating LDR is,where Psat and Plow are the maximum and minimum light intensities,respectively,at which the photocurrent deviates from the linear range. Here,the LDR of the heterojunction is around 34 dB,which limited by the varying power range of illumination of laser.
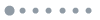
Figure 4.The photoelectric properties at room temperature:(a) The output characteristic curve of the HgTe/ZnO heterojunction detector under 520 nm laser illumination with power varying from 0 to 9 W cm-2;(b) The linear dynamic response range of the HgTe/ZnO heterojunction detector at 0 V;(c) The transient response of the HgTe/ZnO heterojunction detector at 0 V;(d) The response bandwidth of the HgTe/ZnO heterojunction detector
In the realm of photodetection,a short response time indicates the device's ability to quickly adapt to changes in light signals. Typically,response time refers to the time needed for excess carriers to recombine and for the device to recover from both shallow- and deep-level defects. Figure 4(c) illustrates that the falling and rising times,measured between 10% and 90% of the maximum photocurrent,are 455 μs and 410 μs,respectively. In addition,in the self-powered state,the -3 dB bandwidth is 1800 Hz as shown in Figure 4(d).
Further characterization tests were conducted on our fabricated devices at the temperature of liquid nitrogen cooling(80 K). Figure 5(a) illustrates the device's J-V curves in dark state and under illumination of infrared laser at 80 K. It is observed that our device achieves a high rectification ratio close to three orders of magnitude in the dark state. This indicates a substantial enhancement in device performance,emphasizing the effectiveness of the design and materials used in achieving efficient charge separation and rectification in low-temperature conditions. However,for the rectifying characteristics of the device,from the high-temperature n-p junction to the low-temperature p-n junction,this is all attributed to the change in the position of the material's energy bands with temperature[24-25]. At 1 V bias,the dark current density is as low as 5.23 nA cm-2,which is nearly four orders of magnitude lower compared to under 1625 nm laser illumination. This is significantly lower than the dark current reported for most devices based on HgTe CQDs,as summarized in Table 1. Notably,the dark current remains almost unchanged within the voltage range of -2 V to 1 V,attributing to the excellent interface between layers and the role of the ZnO layer as an effective hole-barrier layer. It efficiently transports electrons while blocking holes over a wide range of up to 3 V,which is crucial for real-world applications,ensuring device functionality is not affected by minor external voltage fluctuations
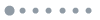
Figure 5.The photoelectric properties at low temperatures:(a) The output characteristic curves of the HgTe/ZnO heterojunction detector at 80 K under dark conditions and under illumination by a 1 625 nm laser;(b) The relationship between current noise and frequency for the HgTe/ZnO heterojunction detector at 80 K with a 1 V bias voltage;(c) The variable temperature output characteristic curves of the HgTe/ZnO heterojunction detector in the dark state;(d) The Arrhenius fitting graph of the HgTe/ZnO heterojunction detector under a bias voltage of 1 V
The noise level of the device is also an important indicator for the practical application of photodetectors. Our device was encapsulated in a Dewar,and the relationship between current noise and frequency under a 1 V bias was tested using a preamplifier and spectrum analyzer,as shown in Figure 5(b). At lower frequencies,the current noise decreases with increasing frequency due to the predominant 1/f noise. At higher frequencies(>10 kHz),the current noise stabilizes around 2×10-17 A Hz-1/2. Extracting data from Figure 5a,with a device area of 100 μm × 100 μm,we obtain a dark current value of 5.23×10-13 A under a 1 V bias,and a photocurrent value of 1.44×10-9 A under 1 625 nm laser illumination at 90 μW power. The net photocurrent,calculated as ,equals 1.44×10-9 A. The responsivity of the photodetector,defined as ,is calculated to be 16 μA W-1. The specific detectivity,an important metric for photodetectors,is defined by the formula,where A is the device area and is the root mean square current noise at a bandwidth of 1 Hz. The specific detectivity of our device is calculated to be 8×109 cm Hz1/2 W-1.
Understanding the mechanism of dark current is crucial for photodetectors. We tested the relationship between dark current and temperature,ranging from 80 K to 280 K,as shown in Figure 5(c). As the temperature decreases,the device's dark current gradually reduces. This is mainly due to the logarithm of the thermal carrier density ni being directly proportional to the reciprocal of the temperature ,i.e.,[26]. As the temperature decreases,the thermal carrier density ni exponentially decreases with the increase of . Additionally,at low temperatures,some defects in the quantum dots are frozen,reducing the possibility of non-radiative recombination and increasing the carrier lifetime,thereby leading to a reduction in dark current. The relationship between the detector's dark current and temperature can be expressed as: ,where C is a temperature-independent factor,K is the Boltzmann constant,and Ea is the activation energy obtained from the Arrhenius fit of the temperature-dependent dark current. The Arrhenius fit,as shown in Figure 5(d),yields an activation energy Ea of 0.315 eV above the crossover temperature Tc=180 K,slightly less than the bandgap of HgTe CQDs,indicating that the dark current at higher temperatures is primarily dominated by diffusion current.
3 Conclusions
In summary,we have successfully developed a HgTe CQDs and ZnO QDs heterojunction photodetector,which showcases exceptional rectification characteristics similar to those observed in p-n junctions. The superior performance of this heterojunction photodetector is primarily due to the meticulous preparation of each thin film layer,ensuring uniformity,and the seamless integration at the interfaces between these layers. This meticulous fabrication process has yielded a device with excellent optoelectronic properties and a robust response to photonic stimuli. Tested under ambient conditions with a 520 nm laser,it demonstrated fast response and wide operational range,making it versatile for optical sensing and imaging. At low temperatures(80 K),it showed high sensitivity and low noise,ideal for precision applications. Variable temperature tests revealed its stable performance at higher temperatures due to diffusion-driven dark current,suggesting broad environmental adaptability. The simple integration method also hints at potential for easy scalability and complementary metal oxide semiconductor compatibility,promising for commercial high-tech applications and bridging lab research with practical use.