1. INTRODUCTION
As a novel all-solid-state beam steering technology, an optical phased array (OPA) features compact size, high reliability, fast speed, and low cost [1,2]. OPA is considered to be an effective technology for many applications, including light detection and ranging (LIDAR) [3], holographic projection [4], and free-space optical communication [5]. Thanks to the mature complementary metal oxide semiconductor (CMOS) process platform, many high-performance on-chip OPAs have been proposed and demonstrated [6–9], for example, aliasing-free 180° beam steering [10,11], less than 0.1° beam width [12], and more than 1000 array elements scale [3]. However, we can notice that most of the reported OPA chips are based on thermo-optic effects to realize phase modulation among the array elements, resulting in relatively low beam steering speeds and limiting their practical applications.
Many different modulation mechanisms and material platforms are used to enhance the operating bandwidth of on-chip OPAs. The free-carrier plasma dispersion effect can provide high-speed electro-optical (EO) modulators for silicon photonics. Several OPAs based on carrier injection or carrier depletion type modulators have been proposed and realized with operating bandwidths of hundreds of MHz [13,14]. Though EO silicon modulators have excellent integration and scalability, the carrier will introduce significant additional absorption loss that increases the light source burden and leads to degradation of the far-field distribution of the OPAs. EO polymers have relatively large electro-optic coefficients, enabling ultra-high bandwidth phase modulators. EO-polymer-based OPAs also have demonstrated operating rates of the order of MHz, but the stability of the polymers has yet to be proven [15]. More recently, high-speed and stable OPAs based on an indium phosphide (InP) platform have been demonstrated, which is CMOS incompatible and also has the issue of additional loss associated with modulation [16–18]. More importantly, we can also notice that almost all of these high-speed OPAs mentioned above have a bandwidth of less than GHz.
Among these EO material platforms, thin-film lithium niobate (TFLN) offers significant advantages such as a high Pockels effect, tight optical field confinement capability, and low propagation loss [19]. TFLN modulators have demonstrated pure phase modulation with CMOS-compatible drive voltages and ultra-high bandwidths [20–22]. In addition, the wide transparency window and high power threshold of TFLN offer great potential for broadening the optical band and operating distance of OPAs. Therefore, the TFLN platform has great potential for realizing high-performance OPA chips. Although some TFLN OPAs have been reported previously [23–25], the scanning range and operating bandwidth are still small, due to the antenna arrangement and RC-limited electrode design.
Sign up for Photonics Research TOC Get the latest issue of Advanced Photonics delivered right to you!Sign up now
In this paper, we propose and demonstrate a TFLN-based 16-channel OPA with high speed and a large field of view (FOV). A traveling-wave modulator array is used to achieve high-speed pure-phase modulation. An aperiodic waveguide array is used to suppress the grating lobes of far-field beams, which enables a large FOV and small beam width. Meanwhile, to avoid crosstalk between antennas, a slab grating is used as the emitter. The experimentally demonstrated modulators have a half-wave voltage () of 6.42 V and a modulation bandwidth of 2.5 GHz. 2D beam scanning with a FOV was realized by combining phase tuning and wavelength scanning. The beam width is in the phase tuning direction () and the wavelength scanning direction (), respectively.
2. DESIGN AND OPTIMIZATION
Figure 1(a) shows the schematic of the proposed high-speed OPA, which mainly consists of a four-stage cascaded multimode interference (MMI) splitter tree, traveling-wave modulator array, and aperiodic waveguide array combined with a slab grating antenna. As shown in the cross-sectional view of Fig. 1(b), the -cut TFLN platform with a 600 nm thick lithium niobate (LN) layer and 2 μm buried oxide (BOX) layer is considered in our work. The device is covered with an 800 nm thick silica cladding. Considering the modulation efficiency of EO modulators and the complexity of fabrication, all waveguides and gratings have the same etching depth of 300 nm. The input laser is coupled to the chip through a grating coupler and then divided into 16 channels with the MMI splitter tree. The modulator array provides independent phase control for each channel, and GS coplanar transmission lines are placed on both sides of the waveguide to drive the EO modulators. Figure 1(c) shows the mode of the waveguide in the modulation region and the distribution of the static electrical field. The output of the modulators is fed into the same slab grating antenna for in-plane interference steering as well as out-of-plane radiation, as shown in Fig. 1(d).
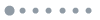
Figure 1.(a) Schematic of the proposed TFLN OPA. (b) Cross-sectional view of the device. (c) Simulated optical mode field and static electrical field distribution in the modulation region. (d) Optical microscope image of the fabricated slab grating antenna.
The modulator array is the key component for realizing high-speed TFLN OPA. In order to utilize the strongest EO coefficient of the LN crystal, the waveguide in the modulation region is transmitted along the direction of the crystal axis. The waveguide width is 1 μm, and the electrode gap is chosen to be 4 μm to maximize the modulation efficiency while ensuring the optical absorption loss of the metal electrodes is negligible (). The calculated half-wave voltage length product () is , which is slightly larger than the typical value reported for TFLN modulators, mainly due to the upper cladding weakening the overlap of the electric and optical fields. The bandwidth of a traveling-wave modulator depends on the impedance matching, velocity matching between microwave and optical signals, and microwave losses [21]. The signal width and the ground width are optimally chosen as 40 μm and 100 μm, respectively. The electrode thickness is 900 nm. Figure 2(a) shows the distribution of the microwave field at the radio frequency (RF) of 20 GHz. The calculated results of the characteristic impedance, microwave index, and microwave loss with microwave frequency are shown in Figs. 2(b)–2(d). Here we choose the length of the modulation region to be 1 cm, and the theoretical modulation bandwidth can be greater than 50 GHz.
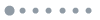
Figure 2.(a) Simulated microwave mode profile. Calculated frequency dependence of (b) characteristic impedance, (c) microwave index, and (d) microwave loss.
Compared with a silicon waveguide, a TFLN waveguide has weaker optical field confinement as well as wider waveguide width, making it difficult to realize a dense waveguide array, which will lead to a limited FOV for TFLN OPAs. Here, we utilize an aperiodic array to suppress the grating lobes. A genetic algorithm is used to optimize the array element spacing over a range from 4 μm to 15 μm. Figure 3(a) shows the simulation results of the far-field distribution of the optimized aperiodic array. The whole aperture size of the waveguide array is 114 μm, which corresponds to a far-field beam width of 0.7°. As shown in Fig. 3(a), we find that the sidelobe suppression ratio (SLSR) is about 9–5.3 dB over a beam steering range of 50°. It should be noted that the relatively low SLSR is mainly limited by the scale of the array elements. For example, the SLSR of greater than 9 dB can be achieved over a beam steering range of 60° when the number of channels for OPA is increased to 64. A conventional waveguide grating antenna will conduct light along the shallowly etched slab layer to neighboring antenna elements, resulting in significant crosstalk. To solve this problem, we use only one slab grating antenna instead of an antenna array. When the waveguide mode propagates into the slab grating, it changes to a divergent slab mode for in-plane interference steering as well as out-of-plane radiation through the grating. The slab grating is designed as a trapezoidal shape, and the tilt angle is chosen to be 15°, which is larger than the in-plane divergence angle of the slab mode, so that more than 90% of the optical field power can be transmitted forward freely without being affected by the reflection of the sidewalls. Figure 3(b) shows the beam steering angle for different wavelengths, corresponding to a scanning efficiency of 0.076(°)/nm.
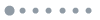
Figure 3.(a) Calculated far-field distribution of the proposed OPA in the phase tuning direction. (b) Calculated steering angle varying with wavelength in the wavelength tuning direction.
3. FABRICATION AND CHARACTERIZATION
The proposed TFLN OPA was patterned by electron-beam lithography (EBL), followed by the inductively coupled plasma reactive ion etching (ICP-RIE) process. Then 800 nm thick silica upper cladding layer was deposited using plasma enhanced chemical vapor deposition (PECVD). Finally, 900 nm thick coplanar waveguide electrodes were fabricated using a lift-off process. Figure 4(a) is the microscope image of the fabricated OPA chip, which includes the proposed OPA as well as a reference Mach–Zehnder interferometer (MZI) used to characterize the EO modulator. Figure 4(b) shows the image of the electrical and optical packaged chip. Pads for all modulators on the chip are bonded to the print circuit board (PCB) via gold wires and then connected to the voltage source and the load resistors (). A fiber array was also packaged on the chip for optical characterization. By characterizing the losses of the unit components, we estimated that the total insertion loss of the OPA chip before the antenna emission is about 9.95 dB, which should be further optimized.
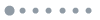
Figure 4.(a) Optical microscope image of the fabricated chip. (b) Image of electrical and optical packaged chip.
We measured the and the bandwidth of the traveling-wave modulator first. As shown in Fig. 5(a), the lightwave of 1550 nm wavelength is coupled into the reference MZI after passing through the fiber polarization controller (FPC). A 10 kHz triangle signal generated by the arbitrary function generator (AFG) drives one of the two arms of the MZI. The modulated optical signal is detected by the oscilloscope (DSO) via a photodetector (PD). Figure 5(b) shows the variation of normalized output light intensity with driving voltage. It can be found that the is , which is in good agreement with the simulation results. We also measured the small-signal EO S-parameters of the modulator using the vector network analyzer (VNA), as shown in Fig. 5(c). Figure 5(d) shows the measured EO response () of the MZI modulator, where the 3 dB modulation bandwidth is about 2.5 GHz. Such modulation bandwidth may be mainly limited by fabrication errors of the large-scale modulator array and the electrical packaging of the OPA chip. Therefore, in order to realize high-speed OPAs, an advanced fabrication process and electrical packaging techniques are also crucial. For a traveling-wave electrode modulator, the energy consumption is about , where is the operating bandwidth, and is the load resistance [20]. When the device operates at 2.5 GHz, each modulator corresponds to a power consumption of .
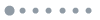
Figure 5.(a) Schematic of the measurement setup for . (b) Measured optical transmission response as a function of the applied voltage. (c) Schematic of the measurement setup for the small-signal EO response. (d) Measured EO of the MZI modulator.
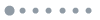
Figure 6.Schematic diagram of the far-field characterization setup.
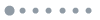
Figure 7.(a) Image of far-field distribution and (b) measured optical power distribution versus angle in the phase tuning direction. (c) Image of far-field distribution and (d) measured optical power distribution versus angle in the wavelength scanning direction. (e) Measured beam width.
4. CONCLUSION
In conclusion, we propose and demonstrate a 16-channel high-speed (GHz) TFLN OPA without grating lobes, for the first time, to the best of our knowledge. By using traveling-wave modulators and an optimized aperiodic array design, we experimentally achieve a modulation bandwidth of 2.5 GHz, an FOV of , and a beam width of in the phase tuning direction and the wavelength scanning direction, respectively. In the future, we will further increase the scale of the array elements and utilize grating antennas of weak radiation strength to improve the performance of SLSR as well as the beam width. In addition, antennas with wide radiation envelopes, such as chain grating antennas, and antennas with metasurface lenses, can be used to further enlarge the FOV [6,30]. We believe that such device will find its applications in high-speed holographic projection, free-space optical switching, and so on.