1. Introduction
Biological detection plays a vital role in modern life[1,2], with fiber optic sensing emerging as a highly sensitive and widely researched detection method[2]. The light source, as the core component of these systems, is critical in determining the performance of the sensing system. Tunable mode-locked fiber lasers (TMLFLs) are a valuable light source, providing high-quality pulsed light over a wide wavelength range and offering features such as flexible wavelength tuning, controllable pulse width, and stable power output[3–6]. These characteristics make TMLFLs highly suitable not only for sensing technology[3] but also for medical imaging[4], spectroscopy[5], and industrial processing[6].
Two commonly used passive mode-locking techniques are widely employed in the field of ultrafast optics to generate ultrashort pulses. The first method involves material-based saturable absorber (SA) mode-locking[7–10], including commercial semiconductor saturable absorber mirrors (SESAMs)[9] and low-dimensional nanomaterials (such as graphene[11], topological materials[7], and quantum dots[12]). Commercial SESAMs and various types of low-dimensional nanomaterials have been widely used in passively mode-locked fiber lasers, ranging from the visible light band to the mid-infrared band. Their advantages include high stability and low mode-locking threshold. The second technique is based on the fiber Kerr effect, such as the nonlinear polarization rotation (NPR) technique[13–17]. Typical components for an NPR-based fiber laser include polarization-dependent isolators[13], a polarization beam splitter[16], 45° tilted gratings[15], and polarization-dependent gratings[17]. Thanks to its high modulation depth and fast response time, NPR mode-locking technology does not require additional nonlinear components and can be effectively combined with pulse-shaping mechanisms under various dispersion conditions, making it the most widely used method for generating picoseconds and sub-hundred-femtosecond pulses in fiber lasers. To enable continuous wavelength tunability of mode-locked lasers, it is necessary to introduce a filter into the laser cavity to select the laser operation wavelength. TMLMLs employing commercial tunable bandpass filters have been demonstrated in various lasers, offering an effective approach to achieving broadband wavelength tuning[18]. However, commercial tunable filters face several limitations in fiber lasers. Their bulky size can hinder integration into compact systems, while high-performance models are often expensive, which increases the overall system cost. Moreover, these filters can introduce significant insertion loss, affecting the efficiency and stability of the laser.
In contrast, all-fiber devices present a more practical solution to these challenges. They offer several advantages such as compactness, better integration with fiber-based systems, lower insertion loss, and reduced cost. Typical all-fiber filters used in mode-locked systems include birefringence filters[19], interference filters[20,21], micro/nano optical fibers[22], fiber Bragg gratings (FBGs)[9], and long-period fiber gratings (LPFGs)[10,23]. Among these, LPFG-based devices offer unique multifunctional capabilities. LPFGs not only act as effective wavelength-selective elements[10,23–25] but also contribute to polarization selectivity within the laser cavity[17,26,27]. By leveraging these properties, LPFG-based solutions directly address the limitations of commercial tunable filters, offering a compact, cost-effective, and versatile alternative. Moreover, the wavelengths of LPFGs can be tuned using various mechanisms such as thermal tuning[23], mechanical strain tuning[9], curvature tuning[24], microfluidic tuning[25], and optical tuning[10], making them an excellent choice for achieving tunable laser output in a wide range of applications.
Sign up for Chinese Optics Letters TOC Get the latest issue of Advanced Photonics delivered right to you!Sign up now
In this work, we demonstrate an erbium-doped fiber ring-cavity laser using a multifunctional LPFG. The incorporation of an intracavity LPFG offers unique advantages, functioning simultaneously as an all-fiber polarization-selective element and wavelength-selective element. This LPFG-based ultrafast fiber laser configuration not only produces stable pulse output by adjusting the polarization state but also enables easy manipulation of the laser operation wavelength through thermal tuning of the LPFG within the cavity.
2. Fabrication of Multifunctional LPFG
Standard single-mode fiber (SMF)-based LPFG allows efficient coupling of light from the core mode to the cladding mode, which leads to the attenuation of specific wavelengths in the transmission spectrum. The resonance wavelength can be described from the phase-matching condition: , where is the resonant wavelength where light coupling occurs between the core mode and the th order cladding mode. and are the core and cladding mode effective indices, respectively, which are determined by the waveguide structure and the material refractive index. is the grating period, which can be flexibly adjusted during the grating design process, and are the orthogonal polarization directions. Figure 1(a) shows the schematic diagram of the LPFG. When an optical fiber exhibits large birefringence, it means that the fiber has a significant difference in the refractive indices along two orthogonal axes, leading to the separation of resonant wavelengths in the transmission spectrum and a high polarization-dependent loss (PDL) of the grating. The orthogonal polarization spectra of the LPFG can be measured using a polarization controller (PC) and a polarization beam splitter (PBS), as shown in Fig. 1(b).
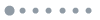
Figure 1.Schematic diagram of the (a) LPFG and (b) polarization-dependent spectral measurement system.
In this experiment, a grating with a period of 600 µm and a period number of 30 was inscribed in the standard SMF-28e fiber using a commercial -laser splicer (LZM-100, AFL Fujikura, Japan). The splicer is equipped with a pair of rotators and two movement motors, enabling stepwise twisting of the fiber during the laser heating process; the other parameter of the grating inscription has been given in our previous work[28]. This technique induces strong asymmetric refractive index perturbation in the fiber, enhancing birefringence and resulting in high PDL. The transmission spectrum of the grating is measured using a broadband low-polarization light source (FL-ASE, FiberLake, China) and an optical spectrum analyzer (OSA, AQ6370D, Yokogawa, Japan). Figure 2(a) shows the measured transmission spectrum of the grating at an OSA resolution of 1 nm, with the two resonance dips corresponding to (left dip) and (right dip) cladding modes. The grating facilitates the fundamental mode to the asymmetric cladding mode coupling in the C-band, achieving a transmission of at 1563.8 nm with an insertion loss of only 0.14 dB. The results indicated that the inscribed grating exhibits both attenuation and wavelength selection characteristics. Additionally, a commercial rotatable polarizer and PC were inserted between the light source and the grating to measure the polarization spectra of the grating. Figure 2(b) shows the measured two orthogonal polarization spectra (top) and calculated PDL spectrum (bottom) in the wavelength range of 1530–1600 nm. The grating achieves a maximum PDL of 21 dB at 1564.5 nm, with an optical attenuation rate of 22.39 dB at the same wavelength. Conversely, at a wavelength of 1547.0 nm, the PDL and attenuation rate of the grating are measured to be 0.76 and 1.21 dB, respectively. The results indicate that the LPFG can serve as an effective polarization-selective in a wider wavelength range. Due to the high sensitivity of the cladding mode on ambient temperature, the temperature response of the was investigated experimentally. The transmission spectrum and PDL spectrum of the grating exhibit redshifts at different temperatures [see Figs. 3(a) and 3(c)]. The resonant wavelength shift of the grating exhibits good linearity with temperature within the range of 25°C–105°C, with a dip showing a temperature sensitivity of 100.84 pm/°C [see Fig. 3(b)]. Moreover, the maximum PDL of the grating is more than 15 dB when the temperature varies within the range of 25°C–105°C [see Fig. 3(d)]. It is important to note that the actual maximum PDL value of the grating should be greater than the measured value due to OSA accuracy limitations and measurement errors. Therefore, through thermal tuning, the grating can function as a tunable wavelength- and polarization-selective element.
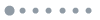
Figure 2.(a) Measured transmission spectrum of the LPFG with a period of 600 µm; (b) measured loss spectrum, orthogonal polarization spectra, and PDL in the wavelength range of 1530–1600 nm.
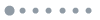
Figure 3.(a) Transmission spectra and (b) PDL spectra of the LPFG as temperature increases; (c) resonance wavelength shift and (d) maximum PDL of the LPFG as a function of temperature.
3. Multifunctional LPFG-based Mode-locked Laser
Unlike conventional saturable absorbers like graphene or SESAMs, which rely on material absorption[9,11], the LPFG achieves mode locking through its polarization-selective properties and the NPR effect[17]. It works with intracavity PCs to induce nonlinear polarization evolution. As intracavity light intensity increases, the polarization state changes, allowing specific polarization components to be selectively coupled. This creates an intensity-dependent loss that mimics the behavior of a saturable absorber for mode locking. As a wavelength-selective element, the grating is crucial for filtering specific wavelengths within the laser cavity, enabling mode locking at a minimum loss position. The schematic diagram of the LPFG-based laser is shown in Fig. 4, with the inset illustrating the structure of the LPFG. The total cavity length of the laser is about 14.07 m, which contains a 0.87 m length of erbium-doped fiber (EDF, OFS EDF80, USA) and 13.2 m length SMF. The group velocity dispersions of the two different types of fiber at 1550 nm are and , respectively. Consequently, the total net cavity dispersion of the laser was estimated to be approximately , indicating that the laser operates in the negative dispersion region. The gain medium is pumped by a 980 nm laser diode (LD, MChlight Co., Ltd., China) and is coupled into the cavity through a 980/1550 nm single-mode wavelength-division multiplexer (WDM). A polarization-independent isolator (PI-ISO) ensures unidirectional laser operation within the cavity. A multifunctional LPFG is inserted between two PCs to function as an equivalent saturable absorber, while a 3:7 fiber coupler with 30% output is employed to couple out the light. Furthermore, the resonant wavelength of the LPFG can be accurately controlled through a thermo-electric cooler (TEC), facilitating the achievement of wavelength tunability in the mode-locked laser.
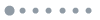
Figure 4.Schematic diagram of the mode-locked fiber laser based on LPFG.
In a multifunctional LPFG-based ring-cavity laser, by precisely adjusting the polarization state within the cavity, a stable self-starting fundamental frequency laser mode-locked pulse was achieved at a minimum pump power of 65 mW. Then, by increasing the pump power to 86 mW, stable single-soliton mode-locked pulses can be maintained. The fundamental frequency laser output characteristics were recorded at a pump power of 82 mW (see Fig. 5). The stable pulse spectrum was measured using an OSA. Figure 5(a) shows a typical soliton mode-locked pulse spectrum with Kelly sidebands, which is a typical feature of traditional soliton fiber lasers. The central wavelength and 3 dB bandwidth of the output spectrum are 1546.26 and 4.6 nm, respectively. The output pulse train of the laser was observed by a 500 MHz digital oscilloscope (DS4050, RIGOL, China) together with a 10 GHz photodetector (PD1040-D, OVLINK, China). The measured pulse train with a pulse interval of about 68 ns [see Fig. 5(b)] corresponds to a repetition frequency of about 14.7 MHz and a laser cavity length of 14.07 m. The inset in Fig. 5(b) illustrates the pulse sequence within a 12 µs time window, demonstrating that stable mode locking has been successfully achieved. Additionally, the radio frequency (RF) spectrum was captured by an electrical spectrum analyzer (ESA, RS-FSV3000, Rohde & Schwarz, Germany), at a 10 kHz span and a 100 Hz resolution bandwidth, with the signal-to-noise ratio (SNR) of the output pulse up to 70.1 dB [see Fig. 5(c)]. The inset picture shows the RF spectrum recorded over a 2 GHz span with a resolution of 100 kHz, also indicating the stability of the laser. The pulse autocorrelation (AC) trace was recorded using an autocorrelator (PulseCheck SM1600, APE, Germany). The output pulse exhibited a full width at half-maximum (FWHM) of 1060 fs, and the actual pulse width, fitted with a function, was 687 fs [see Fig. 5(d)]. This corresponds to a time bandwidth product (TBP) of 0.3963, indicating some chirp in the pulses. The laser produced an average output power of 8.1 mW at a pump power of 86 mW, corresponding to a pulse energy of approximately 0.55 nJ.
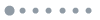
Figure 5.Output characteristics of the LPFG-based fundamental frequency mode-locked operation of the laser. (a) Optical spectrum; (b) output pulse train; (c) repetition rate; (d) AC trace.
By increasing the pump power and adjusting the polarization state, the laser operating at the single-soliton pulses will convert to multisoliton pulses. As the pump power further increases, higher-order harmonic pulses are observed. Harmonic pulses result from long-range soliton interaction; these interactions are typically influenced by dispersion and nonlinearity, which can cause phase synchronization between solitons and lead to the generation of multiple pulses per round trip. Figure 6(a) shows the pulse train of different harmonic orders measured, including the 5th, 11th, 17th, 22nd, 32nd, 40th, 44th, and 50th harmonics. The high-order harmonic pulse is primarily stabilized through soliton interaction dynamics, along with the contributions of intracavity dispersion and nonlinearity. The maximum repetition rate reached 733.8 MHz, the harmonic order could be further improved by increasing the pump power. We measured the output characteristics of the 40th-order harmonic under a pump power of 155 mW, including the output spectrum, RF spectrum, and AC. The 40th-order harmonic corresponds to a repetition frequency of 587.04 MHz, and its spectrum has a central wavelength of 1547.05 nm with a 3 dB bandwidth of 4.53 nm [see Fig. 6(b)]. The SNR of the RF spectrum is higher than 60 dB, indicating that the laser is in a stable harmonic mode-locked state [see Fig. 6(c)]. The FWHM was measured to be 863.4 fs. By fitting the AC trace with a function, the pulse duration is estimated to be 559.6 fs (see Fig. 6(d)). The time-bandwidth product (TBP) is calculated as 0.3175, indicating that the output pulse is near-chirp-free. The average output power from the laser is 14.1 mW, corresponding to a pulse energy of about 0.024 nJ.
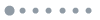
Figure 6.Output characteristics of the LPFG-based harmonic mode-locked operation of the laser. (a) Output pulse train with different orders; (b) optical spectrum; (c) repetition rate; (d) AC trace of the 40th-order harmonic mode locking.
The experimental results show that the LPFG can be used as a polarization-selective element in the NPR mode-locked fiber laser system to achieve stable femtosecond pulse laser output and can serve as an alternative to commercial polarizers or polarization-dependent isolators. Two important points should be noted. First, in our setup, the LPFG exhibits over 99% attenuation at the resonant wavelength, resulting in significant cavity loss that makes mode locking at this wavelength difficult. Therefore, mode locking operates near the edge of the resonance dip (with a loss of ). Additionally, the PDL at the edge of the resonance dip is relatively small. While a lower PDL can make mode locking more challenging, in NPR mode-locked lasers, a PDL of around 1 dB is typically sufficient for stable mode locking[29]. So, the laser still maintains high performance and stable pulse output.
Unlike other polarization-selective elements, such as polarization-dependent isolators[13], 45° tilted gratings[15], and chiral long-period gratings[14], which have broad polarization responses spanning hundreds of nanometers and often generate a mode-locked pulse at random operating wavelengths, the LPFG offers a more controlled approach. Since the attenuation rates of LPFGs for light at different wavelengths near the resonant wavelength vary, pulse laser output with a fixed wavelength can be achieved by balancing the gain and loss within the cavity. The LPFG can function as both a polarization-selective element and a wavelength-selective element, ensuring the generation of pulsed laser output at determined wavelengths. Moreover, the typical features of the LPFG include wavelength tunability, which can be adjusted by factors such as temperature, strain, and surrounding refractive index. This tunability enables the mode-locked laser to be adjustable as well. When the laser operates in a stable harmonic mode-locked state, precise control of the resonant wavelength of the grating using a temperature controller allows for continuous tuning of the central wavelength of the pulse. Figure 7 shows the spectral evolution of the mode-locked laser as the temperature of the LPFG is tuned from 25°C to 105°C. It is important to note that during the spectral tuning process, the pump power and the position of the PC remained unchanged. At a temperature of 25°C, the mode-locked laser outputs the 40th harmonic with a central wavelength of 1546.8 nm. When the temperature increases to 105°C, the central wavelength of the laser spectrum shifts to 1555.2 nm. We linearly fitted the changes in the central wavelength shift of the output spectrum and the output optical power with temperature. The sensitivities are calculated to be 104 pm/°C and 0.015 mW/°C, respectively [see Fig. 7(b)]. The output optical power increases with wavelength due to the rising gain of the EDF in the 1546.8–1555.2 nm range. The laser output power is determined by the balance between gain and loss in the cavity. Furthermore, the mode-locking threshold remains nearly 65 mW over a 10 nm wavelength range. Additionally, due to the varying temperature sensitivity of different order cladding modes of LPFG, or through other grating temperature sensitivity enhancement methods such as coating or adopted dispersion turning point gratings, the sensitivity of the grating resonance dips to temperature can be further improved, thus enhancing the thermal tuning efficiency of the mode-locked laser. The pulse duration and spectral bandwidth of the mode-locked pulse across the temperature range from 25°C to 105°C are shown in Fig. 7(c). The pulse width varies from 559.5 to 694.9 fs, and the spectral width varies between 4.3 and 4.89 nm, with both parameters showing no clear dependence on the lasing wavelength.
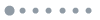
Figure 7.(a) Spectral evolution of the mode-locked fiber laser when the temperature on grating changes from 25°C to 105°C; (b) central wavelength and output power; (c) 3 dB-spectral width and pulse duration of the laser exhibit changes in response to variations in temperature.
All results indicate that the LPFG is a viable alternative device in NPR-based tunable ultrafast lasers. This mechanism enables the LPFG to contribute to the nonlinear saturable absorption effect without the limitations associated with material damage thresholds. Compared to conventional saturable absorbers, such as graphene and other materials, the LPFG-based method offers the advantages in higher power handling, greater wavelength tunability, and enhanced long-term stability, particularly in high-power wavelength-tunable fiber lasers.
4. Conclusion
In this work, we have demonstrated a tunable passively mode-locked fiber laser utilizing a multifunction LPFG as both a polarization-selective element and a wavelength-selective element. By employing a grating with relatively low insertion loss and high PDL, we achieved a stable soliton pulse fiber laser with a high SNR. This LPFG-based NPR mode-locked fiber laser can also operate under harmonic mode-locking conditions by adjusting the pump power and polarization state. Additionally, the LPFG offers wideband wavelength tunability, enabling easy manipulation of the operational wavelength of the laser through thermal tuning of the grating within the cavity. For potential applications, this compact design of LPFG-based laser systems makes them ideal for integration into portable devices, benefiting fields like medical diagnostics by enabling real-time monitoring in minimally invasive procedures. Additionally, the tunability of these systems enhances spectroscopy and sensing applications, allowing for faster and more precise wavelength selection, which is crucial for accurately detecting chemical compounds in industries such as environmental monitoring and food safety.