1. Introduction
The high-power femtosecond (fs) fiber laser, which has the advantage such as compact structure, good long-term stability, and high beam quality, is one of the promising alternatives to conventional solid-state lasers, playing important roles in fields of fundamental research and industry[1–6]. However, due to the transverse mode instability (TMI) and the rich nonlinear effects in the fiber such as self-phase modulation (SPM) and stimulated Raman scattering (SRS), achieving extremely high average power and peak power outputs in an all-fiber architecture is challenging. Thanks to advancements in high-power laser diodes, the evolution of large-mode-area (LMA) fiber, and the development of chirped pulse amplification (CPA) technology[7], high-power fs fiber lasers are capable of generating shorter pulses with increasing average power. In terms of the average power scaling, the performance parameter of a single-channel all-fiber ultrafast laser has reached a new height, and a single-channel ultrafast fiber laser with an MHz repetition rate has achieved nearly kilowatt-level average output power[8–11]. In addition, to break through the power limitations of a single-channel ultrafast fiber laser, scientists have demonstrated the concept of spatiotemporal coherent synthesis of multi-channel ultrafast fiber lasers[12]. In 2006, Mourou et al. proposed the International Coherent Amplification Network Project (ICAN)[13,14], aiming to use tens of thousands of fiber laser arrays to achieve ultra-strong laser pulses with a single pulse energy greater than 10 J, a repetition frequency greater than 10 kHz, and a pulse width shorter than 200 fs, which will be applied to the exploration of particle acceleration. The realization of this major project relies on the development of pulse stacking technology and coherent synthesis technology. Notably, by increasing the repetition rate up to the GHz level, kW average output power can be obtained from a single-channel ultrafast fiber laser[15]. Owing to the significantly lower peak powers, GHz-repetition-rate fs lasers can significantly reduce the nonlinear pulse distortion during high power amplification, and good spatiotemporal frequency characteristics can potentially be guaranteed. Due to the short pulse interval time, the combination of a high power GHz repetition rate ultrafast laser and pulse stacking technology shows great potential in super-large energy and ultra-narrow pulse generation[16,17]. In the context of this important scientific research, further scaling the average output power of GHz-repetition-rate fs fiber laser is technically interesting[18,19].
In this Letter, we report a 2-kW all polarization-maintaining (PM) fiber ultrafast laser system, operating at 1064 nm with a repetition rate of 1.39 GHz. Compared with several previous works reporting on the high average power of fiber lasers, as shown in Table 1, this is the highest average power from all PM fiber fs lasers at 1.0 µm with a single fiber link. The beam quality is measured to be , and the TMI is not presented in this system. The pulse width after compression is measured to be . Further power scaling is limited by the emergence of the SRS, which could be addressed by reducing the overall fiber length of the system or further temporally stretching the pulses. This 2-kW all PM fiber ultrafast laser system could be a promising source for applications like pulse stacking and laser ablation.

Table 1. Key Parameters of the High Average Power Ultrafast Pulsed Lasers
Table 1. Key Parameters of the High Average Power Ultrafast Pulsed Lasers
Repetition rate (MHz) | Average power (W) | Pulse duration (fs) | Beam quality (M2) | Fiber type | Ref. |
---|
69 | 1052 | ∼800 | < 1.2 | non-PM | [8] | 80 | 896 ± 50 | 254 | 1.2 | PM | [20] | 80 | 612 | 863 | 1.29 | PM | [21] | 1.39 × 103 | 1200 | 800 | < 1.1 | PM | [15] | 258 | 1593 | 450 | — | non-PM | [11] | 1.39 × 103 | 2001 | 855 | < 1.2 | PM | This work |
|
2. Experiment and Results
The experimental setup of the all-fiber fs laser system is presented in Fig. 1. The system consists of a passively mode-locked seed laser, a pulse stretcher, four stages of pre-amplifiers, a main amplifier, and a grating-pair compressor. The specifications of the various components used in Fig. 1 are shown in Table 2. The seed laser has a 1.39 GHz repetition rate at a 1064 nm wavelength. After the first pre-amplifier, the pulses are sent to the stretcher consisting of two circulators and two chirped fiber Bragg gratings (each has a group delay dispersion of ), where the pulses are stretched to 300 ps. After the other three stages of pre-amplifiers, the pre-amplified pulses enter the main amplifier for power scaling. The main amplifier is backward co-pumped by a pump module delivering 1100 W power and two pump modules delivering 800 W power, which are coupled into the gain fiber via a 6 + 1 pump-signal combiner. The gain fiber of the main amplifier is an 8-m-long, water-cooled, large-mode-area fiber, characterized by a 20 µm mode field diameter and a 400 µm cladding diameter. A bending diameter of 8 cm was applied to minimize the loss in the fundamental mode but to ensure the adequate suppression of higher-order modes. The pump laser at a wavelength of 976 nm is launched into the main amplifier with a coupling efficiency of 95%, wherein the attenuation is mainly due to the inherent loss of the signal-pump combiner and the fusion-splicing loss. Lastly, the amplified laser is collimated and compressed by the compressor.
Sign up for Chinese Optics Letters TOC Get the latest issue of Advanced Photonics delivered right to you!Sign up now

Table 2. Specifications of the Key Components Used in the Laser System
Table 2. Specifications of the Key Components Used in the Laser System
Component type | Device name | Specification |
---|
Optical fiber device | SPC3 | Pump fiber type | MM 220/242 | Input fiber type | PLMA-GDF-20/400-M | Output fiber type | PLMA-GDF-20/400-M | MFA | Input fiber type | PM-GDF-10/125-M | Output fiber type | PLMA-GDF-20/400-M | CPS | Passive fiber type | PLMA-GDF-20/400-M | End cap | Passive fiber type | PLMA-GDF-20/400-M | SM-LD | Maximum output power | 460 mW | MM-LD | Maximum output power | 27 W | MM-LDs | Maximum output power | 800 W × 2 & 1100 W × 1 | Fiber | YSF | Homemade | | YDF 6/125 | IXF-DF-PM-6-125 | | YDF 10/125 | PM-YDF-10/125-M | | YDF 20/400 | PLMA-YDF-20/400-M |
|
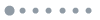
Figure 1.Schematic diagram of the all-fiber fs laser system. All the fibers used in the system are polarization-maintained. SESAM, semiconductor saturable absorber mirror; YSF, Yb-doped single-mode fiber; DF, dielectric film; YDF, Yb-doped double-cladding fiber; WDM, wavelength-division multiplexer; OC, optical coupler; SM-LD, single-mode laser diode; ISO, isolator; CIRs, circulators; CFBGs, chirped fiber Bragg gratings; MM-LDs, multimode laser diodes; MFA, mode field adaptor; CPS, cladding power stripper; SPC, signal-pump combiner.
Figure 2 illustrates the output power as a function of the pump power. At the maximum pump power level of 2612 W, a signal power of up to 2001 W is achieved, corresponding to a power conversion efficiency of 76.6% and an optical slope efficiency of 76.9%. Additionally, the power stability test was conducted at the maximum power of 2001 W within an hour, and the root mean square (RMS) was measured to be 0.16%, as shown in the inset of Fig. 2. It is worth noting that no gain saturation is observed in this case.
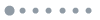
Figure 2.Output power of the 2-kW-average-power main amplifier as a function of the pump power. The inset shows the power stability in an hour.
Figure 3 shows the optical spectra of the main amplifier at different levels of output powers. For comparison, the optical spectrum is also plotted when the pump laser is switched off. The 3-dB bandwidth of the pump-off signal optical spectrum is approximately 4 nm, which is narrowed to about 2.4 nm at the maximum output power of 2001 W, primarily due to the gain-narrowing effect. The inset shows the optical spectrum of the main amplifier in a wider wavelength range of 200 nm, revealing the amplified spontaneous emission (ASE) characteristics and the Raman scattering signal.
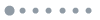
Figure 3.Optical spectra of the main amplifier at different levels of average powers. The inset shows a wider span of 200 nm. ASE, amplified spontaneous emission; SRS, stimulated Raman scattering.
The beam quality of the all PM fiber laser system is shown in Fig. 4, which is measured to be . The spatial beam profile at the highest power is shown in the inset of Fig. 4. Compared with the system described in Ref. [15], the beam quality is slightly degraded, which can be attributed to the increase of the thermal effect as the average power scales up. Figure 5 presents the measured autocorrelation trace at the maximum output power. After compression, the autocorrelation width is determined to be , corresponding to an 855 fs pulse width if a pulse shape is assumed.
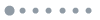
Figure 4.Measurement of the beam quality M2, measured before the compressor at an output power of 2001 W. Inset: beam profile.
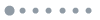
Figure 5.Measurement of the autocorrelation trace (blue) and its hyperbolic secant (sech2) fitting (red), measured at the maximum output power.
3. Conclusion
In conclusion, we demonstrated an all PM fiber ultrafast laser system that delivers 2-kW average power at a wavelength of 1064 nm. This work provides a maximum average output power of up to 2001 W and beam quality of at a repetition rate of 1.39 GHz. This 2-kW-class fs fiber laser system is expected to provide a solid foundation toward achieving a high power (10 kW) all-fiber ultrafast fiber laser system with a minimized footprint.