1. Introduction
Long-wave infrared (LWIR) thermal imaging, capable of providing critical information in scenarios where traditional visual observation is not sufficient, is of great significance in fields such as night vision, medical diagnosis, and aerospace[1-3]. The focus on size, weight, power, price, and performance (SWaP3) optimization in LWIR thermal imaging systems is a key trend in research and development. Metalenses, capable of their subwavelength scale manipulation of wavefront information such as amplitude, phase, and polarization, mark a significant advancement in optical technology[4-10]. They offer the potential to replace or supplement traditional lenses in applications requiring high-precision imaging or in miniature optical systems, attracting significant attention recently.
In 2011, the Capasso group at Harvard University first proposed the concept of a metasurface with anomalous reflection and refraction[11]. Following this, in 2012, a seminal paper on metalenses was published, showcasing the remarkable ability of metalenses to manipulate light at subwavelength scales[12]. In the past decade, great efforts have been made to develop high-efficiency[13-15], achromatic[16,17], and aberration-corrected[18,19] metalenses. Currently, a range of multifunctional imaging techniques and highly integrated devices utilizing metalenses have been developed, particularly in the visible spectrum. Techniques such as spectral tomographic imaging[20], spiral phase contrast imaging[21], extreme depth-of-field imaging[22], spectral light-field imaging[9], and planar wide-angle imaging[23] demonstrate the versatility of metalenses. Moreover, highly integrated devices like metalens endoscopes[24], phase gradient microscopy[25], polarization cameras[26], and pancake meta-optics cameras[27] showcase the broad application prospects of metalens technology. In recent years, LWIR metalenses have shown significant progress, particularly in wide field-of-view imaging[28-32] and broadband achromatic imaging[33-40]. However, to date, the development of LWIR broadband achromatic metalenses with dynamically tunable focus, which are suitable for both coaxial and off-axis applications, remains an important area of research.
In this work, we focus on developing a database of achromatic microstructure units and utilize the particle swarm optimization (PSO) algorithm for the design of dynamic tunable LWIR broadband achromatic metalenses. Given that Si and Ge, commonly used in LWIR metalenses, encounter issues like high refractive indices leading to significant reflection losses and inherent absorption in the LWIR band[41,42], presents a favorable alternative. It boasts a lower refractive index, approximately 2.78 in the LWIR range, and a lower refractive index temperature coefficient, effectively reducing losses and bolstering temperature stability[43]. Moreover, the compatibility of with CMOS processes[44] significantly strengthens its applicability in LWIR metalens applications. These properties of not only improve focusing efficiency and temperature stability but also open up new possibilities for advanced LWIR imaging solutions. Therefore, we employed annular and circular cylindrical microstructures on glass to build the microstructure unit database. The designed LWIR achromatic metalens comprising microstructure units is composed of two parts: a central sub-metalens for near-distance focusing and an outer annular sub-metalens for far-distance focusing. By altering the illumination area, dynamic control of coaxial and off-axis focusing with three dynamic tunable states can be achieved. This work may have potential applications in the design of compact and high-performance optical devices.
Sign up for Chinese Optics Letters TOC Get the latest issue of Advanced Photonics delivered right to you!Sign up now
2. Principle and Design
The design and illustration of an extensive database for constructing a broadband achromatic single-layer metalens, specifically targeting the 9–11 µm wavelength range, are depicted in Fig. 1. Figure 1(a) displays the ideal phase profile for an achromatic metalens with an aperture of 330 µm and a focal length of 500 µm at wavelengths , 10 µm, and 11 µm. The maximum phase difference across these wavelengths, , is 3.4 rad. This spatial phase distribution, which depends on the incident wavelength, can be achieved in principle by subwavelength units of different topologies and structural parameters. In this work, as shown in Figs. 1(b) and 1(c), microstructures made of a subwavelength annular cylinder () or circular cylinder () on substrates are employed. The structural parameters of each antenna are as follows: outer radius (); inner radius (); height (); and period (). In the design of achromatic metalenses, an increased height is beneficial for better dispersion compensation. Therefore, calibrating the microstructure height to align with the designated achromatic wavelength range is a fundamental way to improve optimal performance. Figure 1(d) displays the dispersion compensation ability of microstructure units with a height () of 11 µm when the period () is 5.5 µm. The phase of the transmitted electric field versus the outer radius () and the inner radius () at different incident wavelengths of 9 µm and 11 µm is shown in Fig. 1(d) (left, middle). Figure 1(d) (right) represents the difference between the phases at 9 µm and 11 µm incident wavelengths, indicating the dispersion compensation capability of the 11 µm high microstructure unit database. The black solid line in Fig. 1(d) (right) represents the contour line at a phase compensation value of , showing that the number of microstructures with phase compensation values below 3.4 rad is relatively limited, thus not fulfilling the criteria for database inclusion. Figure 1(e) displays the dispersion compensation ability of the microstructure unit database with a height () of 8 µm when the period () is 5.5 µm. The phase of the transmitted electric field versus the outer radius () and the inner radius () at different incident wavelengths of 9 µm and 11 µm is shown in Fig. 1(e) (left, middle). Figure 1(e) (right) represents the difference between the phases at 9 µm and 11 µm incident wavelengths, indicating the dispersion compensation capability of the 8 µm high microstructure unit database. The black solid line in Fig. 1(e) (right) represents the contour line at a phase compensation value of , showing that the number of microstructures with phase compensation values below 3.4 rad is sufficient, thus fulfilling the criteria for database inclusion. Herein we fixed the height () at 8 µm and constructed the database of broadband achromatic microstructures for the 9–11 µm wavelength range by varying the outer radius () and inner radius ().
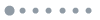
Figure 1.Database of all-As2Se3 microstructure units designed for the 9–11 µm wavelength range. (a) Ideal phase profile of a broadband achromatic metalens for wavelengths λ = 9 µm, 10 µm, 11 µm. (b), (c) Microstructure unit. (d) Dispersion compensation ability of microstructure unit database with a height of 11 µm. (e) Dispersion compensation ability of the microstructure unit database with a height of 8 µm.
3. Results and Discussion
To verify the chromatic correction capability of the developed microstructure unit database, in this section, we utilize this database combined with the PSO algorithm to design an achromatic metalens. The schematic diagram illustrating achromatic focusing is shown in Fig. 2(a). The theoretical phase distribution and realized phase distribution with the PSO algorithm at different wavelengths for a fixed focal length () are shown by the blue curves and red dots in Fig. S1 (Supplementary Material). The structural parameter and transmittance of the microstructures composing the achromatic metalens are shown in Fig. S2 (Supplementary Material). The designed achromatic metalens and an expanded view of the microstructure arrays in the marked area are shown in Fig. 2(b). The output electric field from the achromatic metalens was numerically calculated using finite-difference time-domain (FDTD) simulation software for normal plane-wave incidence. The intensity evolution along the -axis is shown in the first row in Fig. 2(c). The focal length remains almost constant when the incident wavelength changes, indicating the realization of a broadband achromatic focusing in the LWIR region. The second row in Fig. 2(c) shows the electric field intensity distributions at the focal plane (), and the green line represents the intensity distribution at . To further examine the focusing performance of the designed achromatic metalens, the full width at half-maximum (FWHM) and focusing efficiency across five wavelengths for the achromatic metalens are calculated, as shown in Fig. S3 (Supplementary Material). As a comparison, the focusing performance of the chromatic metalens without PSO algorithm optimization at different wavelengths is shown in Fig. 2(d).
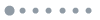
Figure 2.Design and focusing performance of an LWIR broadband achromatic all-As2Se3 metalens. (a) Schematic diagram illustrating achromatic focusing. (b) Designed achromatic metalens and an expanded view of the microstructure arrays in the marked area. (c) Intensity profiles of the achromatic metalens along axial planes at various incident wavelengths. (d) Intensity profiles of the chromatic metalens without PSO algorithm optimization.
After confirming the chromatic correction capability of the microstructure unit database, we now use this database to design an achromatic metalens with dynamic tunable coaxial focusing capability. The schematic diagram illustrating achromatic coaxial tunable focusing under different incident light illumination areas is shown in Fig. 3(a). The achromatic metalens is composed of two parts: a central circular sub-metalens in the central area, designed for encoding the phase for near-distance focusing with focal lengths , and a sub-metalens in the outer annular region, designed for encoding the phase for far-distance focusing with focal length . The theoretical phase distribution and realized phase distribution with the PSO algorithm at different wavelengths are shown by the blue curves and red dots in Fig. S4 (Supplementary Material). The structural parameter and transmittance of the microstructures composing the achromatic metalens are shown in Fig. S5 (Supplementary Material). The designed achromatic metalens and an expanded view of the microstructure arrays in the marked area are shown in Fig. 3(b). The dynamic focusing capability of the metalens under varying incident light illumination areas at different wavelengths is illustrated in Fig. 3(c). Specifically, each row in the figure represents a distinct focusing state: the first row shows near-distance focusing (State 1) achieved by illuminating the inner circular area of the metalens; the second row displays far-distance focusing (State 2) by illuminating its outer annular region; and the third row demonstrates near-far bifocal focusing (State 3) achieved by illuminating the entire metalens. Additionally, each column corresponds to the dynamic control of these three focusing states across five different wavelengths: 9.0 µm, 9.5 µm, 10.0 µm, 10.5 µm, and 11.0 µm, respectively. The focal length in each focus state remains almost constant when the incident wavelength changes, indicating broadband achromatic focusing. The insets in Fig. 3(c) show the corresponding electric field intensity distributions at the focal plane. To further examine the focusing performance of the designed achromatic metalens, we define the focusing efficiency as the ratio between the integral of the electric field intensity within a focal plane region having a diameter D and the electric field intensity’s integral at the output surface of the metalens. Then, the focusing efficiency for these three states can be written as , , , where is 3 times the FWHM, and S1, S2 represent the areas of the two sub-metalenses. The FWHM and focusing efficiency of the achromatic metalens for three states are characterized, as shown in Fig. S6 (Supplementary Material).
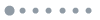
Figure 3.Design and dynamic coaxial focusing performance of an LWIR broadband achromatic all-As2Se3 metalens. (a) Schematic diagram demonstrating dynamic tunable coaxial focusing. (b) Designed achromatic metalens with an expanded view of the microstructure arrays in the marked area. (c) Intensity evolution of each focusing state along the z-axis.
After demonstrating the dynamic tunable coaxial focusing with the designed achromatic metalens, we now aim to adapt this technology for off-axial focusing. The schematic diagram illustrating an achromatic off-axial tunable focusing under different incident light illumination areas is shown in Fig. 4(a). The achromatic metalens is composed of two parts: a central circular sub-metalens with a phase gradient of for near-distance right-deflection focusing with focal length and an outer annular sub-metalens with a phase gradient of for far-distance left-deflection focusing with focal length . The theoretical phase distribution and realized phase distribution with the PSO algorithm at different wavelengths are shown by the blue curves and red dots in Fig. S7 (Supplementary Material). The structural parameter and transmittance of the microstructures composing the achromatic metalens are shown in Fig. S8 (Supplementary Material). The designed achromatic metalens and an expanded view of the microstructure arrays in the marked area are shown in Fig. 4(b). The dynamic focusing capability of the metalens under varying incident light illumination areas at different wavelengths is depicted in Fig. 4(c). Specifically, each row in the figure represents a distinct focusing state: the first row shows near-distance right-deflection focusing (State 1) achieved by illuminating the inner circular area of the metalens; the second row displays far-distance left-deflection focusing (State 2) by illuminating its outer annular region; and the third row demonstrates near-far bifocal focusing (State 3) achieved by illuminating the entire metalens. Additionally, each column corresponds to the dynamic control of these three focusing states across five different wavelengths: 9.0 µm, 9.5 µm, 10.0 µm, 10.5 µm, and 11.0 µm, respectively. The focal length in each focus state remains almost constant when the incident wavelength changes, indicating broadband achromatic focusing. The insets in Fig. 4(c) show the corresponding electric field intensity distributions at the focal plane. To further examine the focusing performance of the designed achromatic metalens, the FWHM and focusing efficiency of the achromatic metalens for three states are featured, as shown in Fig. S9 (Supplementary Material).
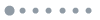
Figure 4.Design and dynamic off-axial focusing performance of an LWIR broadband achromatic all-As2Se3 metalens. (a) Schematic diagram demonstrating the dynamic off-axial focusing. (b) Designed achromatic metalens with an expanded view of the microstructure arrays in the marked area. (c) Intensity evolution of each focusing state along the z-axis.
4. Conclusion
In conclusion, we developed an extensive database of broadband achromatic microstructures for the LWIR spectrum. The chromatic correction capability of the developed dataset was verified by constructing an LWIR broadband achromatic metalens with a fixed focus. This type of LWIR achromatic metalens can be utilized in scenarios that demand high-precision imaging yet require compact optical systems, such as drones and personal portable night vision goggles. Furthermore, using this developed database in conjunction with the PSO algorithm, LWIR metalenses capable of achromatic focusing with three dynamically tunable states, suitable for both coaxial and off-axis focusing, were achieved. This proposed multi-focus metalens is capable of imaging targets at various distances and angles, offering significant utility in scenarios requiring precise imaging across different depths and perspectives, such as obstacle detection and avoidance in autonomous vehicles, navigation of robots in complex environments, detailed observation in medical tomography, and accurate data collection in environmental monitoring and terrain mapping. This study provides a way to realize multifunctional LWIR broadband achromatic metalenses, which may offer the potential for the development of multifunctional LWIR achromatic metalenses with capabilities such as an enhanced depth-of-field and wide field-of-view.