1. Introduction
Plasmonic metal nanostructures can confine optical fields to gaps between metal surfaces with volumes well below the diffraction limit[1–3]. The correspondingly localized electric field enhances light–matter interactions[4–6] within so-called plasmonic nanocavities, enabling weak vibrational spectra or fluorescence of materials to be detected, even down to the single-molecule level[7–9]. Despite progress in developing plasmonic nanostructures with desired configuration to engineer light–matter interaction[10,11], their full potential in the applications in nanophotonic devices[12], quantum optics[13], and molecular science[14], has long been hindered by the limited optical confinement volume (tens of cubic nanometers) in conventional plasmonic nanostructures. However, tip-enhanced Raman spectroscopy (TERS) has resolved the atomic structures of individual molecules[15–17], confirming the possibility of plasmonic confinement to an atomic scale. Recently, several groups have also reported that atomic protrusions (single-adatoms) within the gap of a plasmonic nanojunction can confine light down to the subnanometer scale via the creation of “picocavities”[6,8,18–21].
The direct experimental evidence supporting the formation of the picocavities came from the presence of blinking features in the surface-enhanced Raman scattering (SERS) spectra of spacer molecules in the plasmonic nanocavities[8,18,21]. More specifically, it has been proposed that the interaction between a single adatom at the metal surface and a single molecule would activate initially Raman-forbidden modes in the molecule, resulting in the appearance of transient spectral features, distinct from the constant vibrational modes of molecules in the nanocavities. So far, however, these picocavities were demonstrated to be stabler (lasting several minutes) at cryogenic temperature and become worse (lasting only a few seconds) at room temperature, making their application challenging to the aforementioned areas where selectively controlled and stabilized cavities for demanding light–matter interactions are needed[8,21]. Furthermore, although the model of single-adatom protrusion interacting with a single molecule is highly recommended in the literature, the complex nature of SERS fluctuation requires more detailed insights into the distribution, dynamic evolution, and exact role of the atomic structures in the nanocavity. For example, Shin et al. found that, generally, there existed only one prominent peak in the blinking events in SERS. This can be reasonably ascribed to single-molecule vibrational mode activated by one hotspot induced by a single atomic protrusion located at specific molecular sites[18]. However, in most cases, the SERS fluctuation is always accompanied by the simultaneous appearance of multiple transient spectral features. The simple model of a single hotspot interacting with a single molecule cannot fully explain the activation of these multipeak blinking spectra with random frequencies and fluctuating intensities. The underlying physical mechanism of SERS fluctuation in the plasmonic nanocavities is therefore likely to be a more complex interplay of the local arrangement of atomic features and molecules nearby.
Here, we investigate the intensity and spectral evolution of SERS from biphenyl-1,4-thiol (BPhT) molecules in nanoparticle-on-mirror (NPoM) plasmonic nanocavities at room temperature. We observe both short-duration blinking components lasting for tens to hundreds of milliseconds and, particularly, extremely long-duration blinking components lasting up to minutes, far exceed that commonly observed in the SERS intensity fluctuation in plasmonic nanostructures at room temperature. Each long-duration blinking component shows a contribution from random activation of multipeak features in the SERS spectra. Such multipeak spectral features can be explained by the enhancement of some Raman-allowed vibrational modes and the activation of some Raman-forbidden vibrational modes by atomic hotspots located at different molecular sites.
Sign up for Chinese Optics Letters TOC Get the latest issue of Advanced Photonics delivered right to you!Sign up now
2. Methods
2.1 Sample preparation
The NPoM structures were formed by depositing individual gold (Au) nanoparticles on a thin film of self-assembled monolayer (SAM) of BPhT molecules prepared on an Au film. The flat Au surface was prepared by first depositing 5 nm of a titanium layer at a rate of 0.1 Å/s onto a precleaned double-polished silicon wafer as an adhesion layer and then depositing 100 nm of Au at 1.5 Å/s. The silicon wafer with the Au mirror was immersed in a 1 mM BPhT (Sigma-Aldrich, 97%) solution for 24 h to form a self-assembled monolayer with approximately 1.3 nm thick. About of a colloidal Au nanoparticle suspension ( diameter, optical density in a 1 cm cell) was drop-cast onto the substrate and dried with nitrogen, resulting in randomly dispersed Au nanoparticles on the BPhT-coated Au surface.
2.2 SERS measurements
Raman scattering spectra were carried out based on a home-built scanning confocal microscope. A 785 nm continuous-wave laser was used for excitation. The laser beam was focused by a objective lens with a numerical aperture of onto the sample placed on a piezoelectric nanopositioner (PZT, Tritor, 200/20SG). The SERS signal from molecules within NPoM nanocavities was collected by the same objective and passed through a notch dichroic mirror (Semrock, NFD01-785-) and a notch filter (Semrock, NF03-785 E-25) to block the reflected laser and Rayleigh scattering from the molecules. The SERS signal was then spatially filtered using a 100 µm pinhole and separated by a beam splitter to a single-photon detector (PerkinElmer, SPCM-AQR-15) to measure the SERS intensity fluctuation, and to a spectrometer (Andor SR303i-A with Newton DU971P-BV EMCCD) to measure the spectral fluctuation on both the Stokes and anti-Stokes sidebands.
2.3 DFT calculations
For the density functional theory (DFT) calculations, the BPhT molecule with a thiol sulfur atom bonded to a single Au atom was considered. The most stable conformation features two phenyl rings with a dihedral angle of 39°. The simulated SERS spectra were obtained by first calculating the full Raman tensor via DFT, followed by recalculating under the assumption of local illumination, modeled as a hotspot using a narrow Gaussian beam with a size of 3.5 Å. The vibrational frequencies of all simulated spectra were uniformly scaled by a common factor to align with the experimental peak positions of the three stationary modes at 1080, 1281, and .
2.4 DFS measurements
Dark-field scattering (DFS) spectra of the NPoM structures were measured using a home-built dark-field slit imaging system based on an inverted optical microscope (Olympus, BX53). Briefly, a quartz-tungsten-halogen lamp was used for illumination. The white light was focused by a hollow objective lens (Olympus, , ) on the sample after passing through an aperture with a light stop. The scattering signal from individual NPoM nanocavities collected by the objective was measured by a spectrograph (Princeton Instruments, PI-HRS300) coupled with a CCD camera (ProEM-HS: 1024BX3). The spectra were calibrated by dividing the normalized spectrum of the light source.
2.5 Simulations using COMSOL
To simulate the near-field enhancement and spectral response of the picocavity, a commercial finite-element method (FEM) package [COMSOL Multiphysics 5.6 with the radio frequency (RF) module] was employed. The nanoparticle was modeled as a truncated sphere with a diameter of 120 nm, positioned above a molecular layer with a cross-sectional diameter of 50 nm. The molecular layer was assigned a thickness of 1.3 nm and a refractive index of 1.45. To model the Au film and the silicon substrate beneath the molecular layer, the Au substrate and silicon base were treated as infinite regions. Perfectly matched layers were applied to truncate the computational domain, simulating semi-infinite air and substrate regions to minimize boundary scattering. The refractive index of the silicon substrate was set to 3.48. The single-atomic protrusion of the picocavity was modeled as a hemispheroid with a diameter of 0.3 nm, reflecting the physical dimensions of a single Au atom. In all calculations, the dielectric constant of Au was derived from Johnson and Christy’s experimental data. The structure was illuminated at an oblique angle of 55°, mimicking the experimental incidence angle, and a two-step method was used to compute the scattering field. First, the background field of the structure without the protrusion was calculated. Then the total field, including the single-atom protrusion, was computed based on the background field, and the scattering cross section was obtained using built-in postprocessing functions. To ensure the accuracy and convergence of the results, finer mesh refinement was applied in the gap and protrusion regions.
3. Results and Discussion
Figure 1(a) shows the schematic view of the NPoM construct. The coupling of plasmons in the Au nanoparticle with its image charges in the mirror tightly confines the light field in the gap region, which can thus amplify the SERS signal of the BPhT molecules. Figure 1(b) shows a typical SERS spectrum of BPhT molecules in a nanocavity, where the interaction between the laser and the molecular vibrations generates Raman sidebands at lower (Stokes) and higher (anti-Stokes) frequencies. Three dominant peaks appear on both sides of the spectrum, corresponding to Raman-active molecular vibrations with constant frequencies at (green arrow), (blue arrow), and (red arrow), respectively, reflecting the thermal vibrational populations at room temperature.
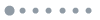
Figure 1.(a) Schematic view of the NPoM plasmonic nanocavities, where Au nanoparticles are separated from an Au film (100 nm thick) by a self-assembled molecular monolayer. The inset illustrates the self-assembled BPhT molecules in the gap region. (b) Typical SERS spectrum of BPhT molecules in an individual plasmonic nanocavity. Arrows indicate three ever-present Raman-active vibrational modes in BPhT molecules: 1080 cm-1 (green arrow), 1281 cm-1 (blue arrow), and 1595 cm-1 (red arrow), respectively. The acquisition binning time is 10 s. (c) and (d) Time series of the anti-Stokes (upper panel) and Stokes (lower panel) spectra from two typical NPoM constructs. Binning time: 10 s. (e) and (f) Time series of the integrated SERS intensity from the two typical NPoM constructs, respectively. Binning time: 10 ms. (g) Statistics of the duration of blinking events observed in 20 plasmonic nanocavities.
In addition to these stationary components existing permanently in SERS, many blinking components appear in the spectra, accompanied by an abrupt increase of the integrated SERS intensity. Figures 1(c) and 1(d) show typical SERS spectral trajectories from two different plasmonic constructs, with the anti-Stokes and Stokes spectra recorded simultaneously. Generally, the blinking events in SERS can be characterized by the appearance of many spurious peaks with randomly shifting frequencies and flickering relative intensities [Fig. 1(c)]. We simultaneously recorded the Raman scattering intensity of molecules in individual constructs by integrating over the scattering signal of the whole spectrum (including both the Stokes and anti-Stokes scattering) with a single-photon detector (10 ms binning time) [Fig. 1(e)]. Whenever blinking components appear, the integrated scattering trajectory shows a significant increase in intensity, far exceeding the baseline that arises from the stationary components [Fig. 1(e)]. Interestingly, we observed sharp protrusions in the integrated scattering intensity of some constructs as shown in Fig. 1(f), whereas no obvious spurious peaks were found in its SERS spectrum [Fig. 1(d)]. Similar fast-flashing components can also be found in Fig. 1(e). By statistically analyzing the duration of each blinking component appears in integrated SERS intensity trajectories of about 20 plasmonic constructs [Fig. 1(g)], we can find that most fast-flashing components as shown in Fig. 1(f) occur over a quite short timescale from tens to hundreds of milliseconds [Fig. 1(g-I)]. Such a short duration for blinking events has commonly been found in the literature. Recent experiments have even demonstrated the detection of SERS intensity fluctuations down to microseconds using high-speed imaging techniques[20,22]. However, many blinking events with duration time up to minutes were observed in our experiment [Fig. 1(g-III)]. Such a long-duration SERS fluctuation, to the best of our knowledge, has not yet been characterized in the work performed at room temperature.
To clarify the possible reason for such a significant difference in the duration time of the blinking components, it is important to first consider the underlying mechanisms of spectral fluctuations in SERS. Previous investigations have shown ubiquitous intensity and spectral fluctuations in a variety of SERS systems under laser irradiation. For instance, in individual metal constructs, the spectral fluctuation in SERS has been assigned to various dynamics, such as rapid molecular adsorption and desorption on the metal surface[9], diffusion of single molecules on the metal surface[23], and laser-induced reconstruction of the metal surface[21,22]. In our case, the BPhT molecules are believed to be densely packed in a self-assembled monolayer prepared upon a long incubation time[24,25]. Moreover, considering the molecules that contribute to the SERS signal are confined in the gap region of the plasmonic nanocavities, it is not feasible to attribute the blinking events to the adsorption/desorption or diffusion of individual molecules in the gap. Another contribution that might have an influence is the blinking of the intrinsic luminescence from Au nanoparticles, which has been observed very recently in plasmonic nanocavities and can be enhanced by the plasmonic resonance[19]. However, it is noteworthy that the energy of the excitation laser (785 nm) used in our experiment is much smaller than the interband absorption from -band to -band of Au which may account for the photoluminescence (PL) emission[26,27]. It is therefore unlikely that PL occurs when Au nanoparticles are excited at such a longer wavelength. Moreover, a clear correlation between the enhanced features on the Stokes side and those on the anti-Stokes side can be found in the spectra [Fig. 1(c)]. This rules out further the possibility that the transient fluctuations in SERS come from the PL of Au.
Recently, Baumgerg et al. proposed that metal surface reconstruction could play a key role in triggering spectral fluctuations in SERS within plasmonic nanocavities[28,29]. Specifically, they suggested that the blinking in SERS spectra results from the random formation and disassembly of single atomic protrusions (thus termed “picocavities”) on the metal surface, which activate previously Raman-inactive modes in single molecules. However, it was generally believed that atomic protrusions were difficult to stabilize at room temperature[8,21]. Considering this, the long-duration blinking events observed in our study at room temperature (as shown in Fig. 2) may indicate the presence of additional dynamic mechanisms. As we will discuss further, instead of the single-atomic protrusion interacting with an individual molecule, we propose that the dynamic evolution of multiple atomic protrusions simultaneously is responsible for the long-duration blinking, while the formation and disassembly of individual atomic protrusions likely cause the transient blinking events with very short durations.
![(a) Time series of the Stokes and anti-Stokes spectra from a typical NPoM nanocavity showing long-duration blinking components. The acquisition time is 10 s. The middle column shows the time series of the integrated SERS intensity from these NPoM constructs, including signals from both Stokes and anti-Stokes. Binning time: 10 ms. (b) The spectra extracted from the time-series SERS spectra at the beginning of the acquisition and at different time points [210, 520, and 620 s as indicated by white arrows in (a)] when blinking features occur. The blue-shaded areas highlight the Raman-active peaks that are ever-present during the measurement, while the orange-shaded areas represent Raman-inactive peaks, which only appear randomly when blinking occurs. The acquisition time for each spectrum is 10 s. (c) DFT calculations of the infrared absorption (left column) and Raman cross section (right column) for a single Au-bound molecule (Au-BPhT) driven by a uniform field. The blue-shaded areas highlight both IR and Raman-active peaks.](/Images/icon/loading.gif)
Figure 2.(a) Time series of the Stokes and anti-Stokes spectra from a typical NPoM nanocavity showing long-duration blinking components. The acquisition time is 10 s. The middle column shows the time series of the integrated SERS intensity from these NPoM constructs, including signals from both Stokes and anti-Stokes. Binning time: 10 ms. (b) The spectra extracted from the time-series SERS spectra at the beginning of the acquisition and at different time points [210, 520, and 620 s as indicated by white arrows in (a)] when blinking features occur. The blue-shaded areas highlight the Raman-active peaks that are ever-present during the measurement, while the orange-shaded areas represent Raman-inactive peaks, which only appear randomly when blinking occurs. The acquisition time for each spectrum is 10 s. (c) DFT calculations of the infrared absorption (left column) and Raman cross section (right column) for a single Au-bound molecule (Au-BPhT) driven by a uniform field. The blue-shaded areas highlight both IR and Raman-active peaks.
To validate this speculation, we analyzed the time evolution of the blinking components in detail. Figure 2(a) shows typical SERS spectral trajectories from a plasmonic construct where long-duration time blinking components up to 3 min appear in both the anti-Stokes and Stokes spectra simultaneously. We extracted the spectra at the initial moment and different time points [(210, 520, and 620 s, indicated by white arrows in Fig. 2(a)] of the blinking components from the time-series SERS spectra. It should be noted that each blinking feature in the SERS can be attributed to the appearance of distinct peaks both on the Stokes and anti-Stokes sides [Fig. 2(b)], whose intensity changes over time, resulting in a significant increase in the integrated SERS intensity trajectory shown in the middle column of Fig. 2(a). By comparing the extracted spectra with the infrared (IR) absorption spectra and Raman spectra simulated by the DFT [Fig. 2(c)], we found that the spectral peaks in SERS can be divided into two sets: A first set of lines can be found both in the simulated IR spectra and Raman spectra at any time [Fig. 2(b), blue shaded]. A clear correlation between these peaks and the intrinsic Raman-allowed vibrational modes of the molecules can be clarified. The second set of lines are normally Raman-inactive [Fig. 2(b), orange shaded]. Some of them can be found in the IR spectra, while others cannot be found either in the simulated IR absorption spectra or in the simulated Raman spectra. Considering the nature of the SERS spectra, the appearance of these anomalous lines indicates that specific vibrational modes in molecules are selectively and randomly activated during laser irradiation, resulting in an increase in the integrated scattering intensity of the plasmonic constructs. By contrast, the SERS intensities of the stationary components remain unaffected by the appearance of the blinking components. This suggests that the stationary components originate from the molecules inside the gap of the plasmonic nanocavity, whereas the blinking components may arise from extremely small hotspots that only significantly enhance the SERS intensity of nearby molecules, leaving the rest of molecules in the nanocavity unaffected. It has been suggested that such tight confinement in an extremely small volume can be realized when atomic-scale structures appear inside the plasmonic gap[21,30]. The ultrasmall localization of light can thus yield strong optical field gradients that switch the Raman selection rules[7,31], enabling previously Raman-inactive modes to become visible.
Theoretically, the activation of the Raman-inactive modes can be simulated by recalculating the SERS spectrum under the assumption of inhomogeneous field illumination at the subnanometer scale. To theoretically reproduce the blinking peaks observed in the SERS spectra, we simulated the Stokes spectra by calculating with a hotspot size of 3.5 Å and various positions relative to a single molecule (See Sec. 2 for details). It can be found that different blinking components observed in the experimental spectrum can only be well reproduced when the hotspot was positioned at a different specific site of the molecule with a different tilt angle [Figs. 3(b) and 3(c)].
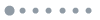
Figure 3.(a) Comparison of the initial moment SERS spectra of BPhT molecules with the spectra at 210 s of laser irradiation. The colored areas highlight four newly emerging vibrational modes observed in the blinking event. (b) Calculated SERS spectra for different hotspot positions relative to the molecule, which reproduce the specific vibrational modes observed in the experiment as shown in (a). The diameter of a hotspot for the simulations is 3.5 Å. (c) Schematic views of the locations of the hotspots (red dots) relative to the atomic positions in the molecule, leading to the corresponding simulations shown in (b).
However, we should note that multiple Raman-inactive peaks and Raman-active peaks appeared simultaneously in one blinking component. This is quite different from the observation of Shin et al.[18], where the majority of SERS spectra displayed only one vibrational peak in the blinking events and could be hardly well explained with the interaction between a single-adatom protrusion and a single molecule proposed by Benz and coworkers[8]. Considering the multipeak features that occurred in a single blinking event observed in our experiment [Fig. 2(b)], it is therefore natural to think that the cooperation of multiple hotspots is required to reproduce the simultaneous activation of multiple Raman-inactive vibrational modes and enhancement of some initially Raman-active modes in molecules. This implies that there can sometimes be several single atomic protrusions interacting at the same time with different sites of a single molecule or each with a different single molecule.
Based on these observations, we believe that the dynamical creation and destruction of these multiple single atomic protrusions results in the activation and fluctuation of multiple vibrational modes in the SERS spectra. On the other hand, due to the dynamic nature of these single adatoms, the simultaneous annihilation of these protrusions will be difficult. Once all these single adatoms disappear, the integrated scattering intensity returns to the baseline level that comes from the stationary components [Fig. 2(a), middle column]. This may explain the much longer duration time of the blinking components observed in our experiment [Fig. 2(a)]. In contrast, we suggest that the transient blinking events with much shorter duration time on the order of microseconds may result from the dynamic creation and disappearance of a single atomic protrusion induced by laser irradiation. As shown in Fig. 4, simulation on the near-field enhancement with a single Au atom protrusion demonstrates that such a protrusion can confine light to a subnanometer volume, increasing the near-field intensity by 6 orders of magnitude. This substantial local electric field enhancement is believed to create strong field gradients, thus breaking the Raman selection rules and activating certain Raman-inactive modes in the molecules within the picocavity[21,31]. The fast dynamic evolution of the single atomic protrusions gives rise to extremely short-duration blinking events [Fig. 1(f)]. Due to the instantaneous characteristics of these picocavities, corresponding features are not observed in the SERS spectra, primarily due to the time resolution limitation (10 s) in our case. If the time resolution of spectral acquisition is high enough while keeping a sufficient signal-to-background ratio, we believe that the spectral fluctuations contributing to the short-duration blinking events can be further characterized. This has been demonstrated recently in the literature, where fluctuations in the microsecond timescales can be characterized based on high-speed acquisition methods[20,22].
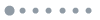
Figure 4.(a) Geometry of the NPoM structure used for simulating the optical response. (b) Near-field enhancement with and without the atomic protrusion, with the white circle highlighting the subnanometer localization of the optical field in the presence of the atomic protrusion. (c) Simulated dark-field scattering spectra for NPoM constructs with or without the atomic protrusion. (d) Dark-field scattering spectra of a representative NPoM construct, recorded before and after 15 min of laser irradiation.
As recent studies highly suggested that it was not the role of light excitation, but rather the thermal heating in generating the atomic featured in metal surface[19,32], the creation of multiple atomic protrusions was possible considering the higher power density () used in our case than that in the previous literature, as we found that the blinking events were more pronounced at larger laser power. To further investigate the possible influence of light-induced atomic protrusions on the geometry of the plasmonic nanocavities, we measured the dark-field scattering spectra of NPoM constructs before and after 15 min of laser irradiation. Despite the persistent blinking during light exposure, the dark-field scattering spectra of NPoM showed no significant changes [as shown in Fig. 4(d)]. This implies that the creation and annihilation of either the single atomic protrusion or the multiple protrusions will not change the geometry of the nanocavities. The far-field scattering spectra primarily depend on the properties of the larger host nanocavity, while the formation of the picocavities is only responsible for the near-field SERS enhancement. Although the formation of the atomic features cannot be characterized by the dark-field scattering spectra of the nanocavity, substantial progress has been made to resolve their locations. Recent experiments performed with superresolution imaging and high-speed imaging have revealed that the SERS fluctuation occurs on the localized () regions of the nanoparticles[22,33]. Characterization of atomic-scale fluctuations requires further improvement in spatial resolution, which is of fundamental scientific and technological interest.
4. Conclusion
In summary, we studied the intensity and spectral blinking behaviors of SERS from BPhT molecules in plasmonic nanocavities and obtained new insights into the nature of atomic features that induced these phenomena. Both extremely long-duration blinking components (lasting up to minutes) and short-duration blinking components on the order of tens to hundreds of milliseconds were found simultaneously in individual plasmonic constructs under ambient conditions. Detailed experimental investigations on the spectral features that contribute to the blinking components demonstrated that multiple vibrational peaks, either Raman-allowed or Raman-forbidden, appear simultaneously in an individual SERS intensity blinking event. The successive and random boost and eventual disappearance of these vibrational modes at different frequencies lead to the existence of long-duration blinking components. Theoretical calculations of the SERS spectrum under inhomogeneous field illumination at the subnanometer scale (simulating the “hotspot”) show that, to reproduce the activation of multipeaks in a blinking component, multiple hotspots interacting with different molecular sites are required. This suggests that there should sometimes be several single atomic protrusions interacting at the same time with different sites of a single molecule or each with a different single molecule. Our results promote the studies of atomic-scale structural changes in plasmonic nanocavities and their role in influencing the SERS fluctuation. However, the formation and position of hotspots are random during the current process. Achieving precise control of the hotspot formation and their position could open up the possibilities for driving unusual light–matter interactions at the nanoscale.