1. Introduction
Human utilization of pigments dates back to ancient times; however, challenges such as unstable color appearance and environmentally unfriendly manufacturing processes have persisted throughout history. Recently, researchers have turned their attention to structural colors, also known as physical colors[1]. These colors are created from physical phenomena such as interference, diffraction, and scattering. They are environmentally friendly, are abundant in raw materials, and can easily create iridescence[2,3]. Therefore, so far, their applications span anti-counterfeiting[4], decorations[5], solar cells[6], and so on.
Properties of structural colors are intricately tied to the structure arrangement and material selection. Typically, once the manufacturing processes are completed, the colors created are fixed and demonstrate long-term stability. In conventional application fields, this characteristic is appealing and can never be too much. However, in dynamic displays where different colors are required, transformation between these colors plays an important part in conveying information, which indeed poses a significant challenge. In 2014, Hosseini et al.[7] designed a reflective and semi-transparent dynamic structure by incorporating a thin layer of (GST) phase-change material between two transparent indium tin oxide (ITO) electrodes. Upon stimulation of an electric pulse, the GST thin film layer crystallized, inducing changes in the refractive index and then in the optical properties of the whole structure. Visually, it is a noticeable change in color. Later in 2016, Ríos et al.[8] introduced another four-layer reflective structure using other phase change materials (PCMs). Considering the fact that the phase state of PCMs determines the optical properties of the structure and distinguishable performance can be achieved in response to specific heat or electric stimuli, such as crystallization or melt-quenching processes, on the picosecond timescale, they explored the potential value of this structure in nano-display technology.
In fact, color change brought by the phase transition of PCMs has introduced new prospects for the usage of structural colors, not only in the field of flexible and dynamic displays[9,10] but also in other fields including smart windows[11] and artificial retinal devices[12]. Commonly used PCMs for dynamically tunable structural colors include GeTe[13], [14,15], and GST[16,17]. While these materials offer exceptional color tunability to film stacks, they concurrently function as absorbing layers, thereby restricting color brightness and saturation. In 2017, Zhang et al.[18,19] developed a new kind of PCM from GST by doping in the Se element. They explored the electrical and optical properties of with different and designed a single-mode SiN waveguide resonator device with (GSST). Thanks to its enhanced optical performance, such as low energy loss and excellent optical contrast, the device was reported to have a significant on/off contrast ratio of 41 dB and an insertion loss of 0.2 dB. Since then, GSST has been used in the design of various optoelectronic devices. However, it is worth noting that the use of GSST as ultrathin optical coatings in the visible range for dynamic structural colors has rarely been studied[20].
Sign up for Chinese Optics Letters TOC Get the latest issue of Advanced Photonics delivered right to you!Sign up now
Here, GSST is introduced as an alternative to traditional PCMs to create dynamically tunable structural colors with excellent color performances. Using an ion-assisted electron beam evaporation method, single-layer thin films made of GSST material with different thicknesses were prepared, and to investigate the optical characteristics changes in visible light brought by the phase transition of this material, annealing experiments were conducted with different conditions. In comparison with traditional PCMs, GSST material retains the property of large optical constant changes, while further reducing its intrinsic absorption. Therefore, a four-layer thin film structure was proposed using this material. Along with the phase change of GSST, its reflection spectra can be dynamically adjusted, resulting in distinguishable colors. Besides, full colors covering a large area in CIE color space and exhibiting high color saturation and brightness can be easily achieved simply by altering the layer thickness. Considering the simple preparation processes of this structure, this scheme is expected to be widely used in various displays, anti-counterfeiting, and artificial retina applications.
2. Results and Discussion
2.1. Structure design and simulation
As mentioned earlier, PCMs are of great importance in the dynamic tunability of structural colors. The essence lies in the refractive index change during the crystallization or melt-quenching processes of these materials. Here, the refractive index (n) and extinction coefficient (k) of the GSST thin film of two states under the ideal deposition conditions are measured and presented in Figs. 1(a) and 1(b). Optical constants of GST material from Ref. [21] are also included for comparison. In the plots, “a” stands for the amorphous state, and “c” represents the crystalline state (c-state). It can be seen that both materials have a large refractive index, more than 3 at 400 nm and increasing with wavelength. Notably, the extinction coefficient of GSST is much lower than that of GST. This means more efficient use of light energy. After stimulation [from Figs. 1(a) to 1(b)], the optical constants of two materials undergo significant changes. The refractive index in the c-state increases more abruptly than in the amorphous state and the extinction coefficient rises obviously throughout the visible range after the phase transition. Different from the refractive index changes of GST, which behave as decreasing at short wavelengths and increasing at long wavelengths, the refractive index of GSST increases from 400 to 800 nm covering the full range. However, overall, the tunability of optical constants for these two materials is comparable, both ensuring dynamic color changes in multi-layered structures. Besides, with lower values in the extinction coefficient of GSST, structures using this material are more likely to reflect colors with high brightness.
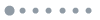
Figure 1.Optical constants of GSST and GST: (a) amorphous state; (b) crystalline state. (c) Multi-layered structure proposed in this paper, sub/Ag/LaTiO3/GSST/LaTiO3/air, and the way it modulates the incident light to dynamically reflect different colors.
In Fig. 1(c), a four-layer structure is illustrated. is sandwiched between a Ag mirror and a GSST thin film layer, forming an asymmetric Fabry–Perot (F–P) cavity. The GSST layer partly reflects the light coming from the bottom to form a localized resonance and also ensures dynamic tunability of the whole structure because of its phase change phenomenon. Besides, in order to improve the color saturation, this F–P cavity is complemented by an anti-reflection (AR) unit made of , which effectively suppresses the sideband reflection by eliminating the impedance mismatches between GSST and incident material (normally air). Here, is chosen because it has a lot of benefits, such as a relatively large refractive index, low absorption, and stable optical properties including resistance to high temperatures and minimal reliance on oxygen[22].
In this multi-layered structure, the AR unit is a crucial component. To demonstrate its function further, we conducted calculations of the admittance trajectory diagrams for the thin film structure both with and without the AR unit. Using their admittance, the reflectance of the film stack can then be computed. The admittance trajectory diagram documented the admittance of the ideally equivalent substance while the multi-layered structure was deposited on the substrate from bottom to top. Thus, taking a closer examination of this reflectance-calculating formula, it is evident that the reflectance is actually the two-dimensional Euclidean distance between the ending point and the (1, 0) point (representing the admittance of air) in the diagram. In Fig. 2 [Figs. 2(a) and 2(d) are red; Figs. 2(b) and 2(e) are green; and Figs. 2(c) and 2(f) are blue], this distance is highlighted by a solid black line. The longer the line, the higher the sideband reflection. From the results, one can see that for structures without the AR unit [Figs. 2(a)–2(c)], the sideband reflection is much higher. Red, green, or blue are all the same.
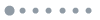
Figure 2.Multi-layered structure: sub/Ag/LaTiO3/GSST/LaTiO3/air. (a)–(c) The admittance trajectory diagram of the red, green, and blue multi-layered structure without the AR unit. (d)–(f) The admittance trajectory diagram of the red, green, and blue multi-layered structure with the AR unit.
The optical properties of this structure were explored further. First, GSST films of different thicknesses were inserted into the structure to investigate the relationship between the phase change layer’s thickness and the color performance and tunability of the film stack. Taking the structure that reflects red colors as an example, the results are shown in Fig. 3(a), where the coordinate axes X, Y, and Z of these two three-dimensional models represent the wavelength (ranging from 400 to 800 nm), the layer thickness of GSST thin film (ranging from 5 to 55 nm), and the reflectance of the entire film stack (%), respectively. The model on the left shows the reflectance spectra when the GSST layer is at the amorphous state, and on the right, it is at the c-state. For both states, an increase in the layer thickness leads to a redshift in the entire spectra and to some decreases of value in the peak reflection. It is important to note that the reflection declines more rapidly at the c-state. Besides, the structure with c-state GSST tends to exhibit a much dimmer background [dashed circles in Fig. 3(a)], which is the essence of high-saturation structural colors. For example, blocks in the middle indicate the colors with varied GSST thicknesses, ranging from 5 to 55 nm. Observations suggest that color blocks on the right, which stand for the c-state, are purer and more authentic. Thus, it is believed that bright and saturated c-state colors can be produced when the phase change thin films are not too thick. Normally, 10–25 nm is appropriate. In this area, there is also a distinct color change between two phase states, representing better color tunability. Second, optical characteristics of the structure with varied thicknesses of two layers (middle layer as d1 and top layer as d2) were also studied, as shown in Fig. 3(b). Specifically, d1 ranges from 40 to 200 nm, d2 ranges from 100 to 300 nm, and the same calculations were performed twice for each phase state of the GSST material. It is observed that the color changes follow the same patterns. Along the positive direction of the vertical coordinate representing d1, the color hue undergoes significant changes. Various colors are produced, including blue, green, yellow, and red, whereas colors created by increasing or decreasing the thickness of d2 remain similar in color hue, except for some improvements in brightness and saturation. Thus, by altering the film thickness of the intermediate layer and matching it with a properly adjusted top layer, a lot of dynamic colors covering the full gamut and with high purity can be easily achieved. Notably, while the GSST layer is at its c-state, various colors are more distinguishable or have larger color contrast. Additionally, in Fig. 3(c), different colors reflected by the same four-layer thin film structure with c-state GSST or GST are plotted in the chromaticity diagram for comparison of color diversity. Apparently, the color range covered using GSST material is wider.
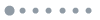
Figure 3.(a) Thickness effect of the GSST layer on the spectra of the structure mentioned above: the x-axis is the wavelength, the y-axis is the GSST thickness, and the z-axis is the reflectance; the color blocks between are equipped with GSST thicknesses of 5, 10, 20, 30, and 55 nm from bottom to top. (b) Thickness effect of LaTiO3 dielectric layers on the colors of the structure mentioned above. (c) Comparison of the color gamut of the same structure mentioned above but with different phase change materials: GSST or GST.
2.2. Phase change characteristics of GSST and experiments
In this study, all thin films were prepared using the ion-assisted electron beam evaporation system due to its numerous advantages, including the adjustable deposition rate, high density, simplicity for large-area preparation, and high film quality. To achieve optimal optical characteristics for the (GSST) PCM, the deposition process was modified, and experiments were conducted to investigate the ideal annealing conditions for its phase transition. All layers were prepared by ion-assisted electron beam evaporation technology. The electron gun was a 270-deg deflected e-gun, which effectively avoided the influence of secondary electron radiation on the quality of the film. The ion source was a Kaufmann high-energy ion source, which ensured the high density of the film and caused the film material to have stable optical constants. The BK7 glass with a diameter of 30 mm was chosen as the substrate, and the substrate was wiped clean with a mixture of alcohol and ethanol before it was placed into the electron beam evaporation chamber. The working vacuum is . The material purity of GSST and used is 99.99%, and the specification is 3–5 mm irregular particles. The purity of the Ag used is 99.999%, and the specification is columnar silver particles. The deposition rates of Ag, , and GSST are 10, 3, and 1 Å/s, respectively. The preparation parameters of the materials used in this paper are shown in Table 1.

Table 1. Material Preparation Parameters
Table 1. Material Preparation Parameters
Material | Work vacuum () | Deposition rate (Å/s) | Ion voltage (V) | Ion beam (mA) |
---|
Ag | 8.0 | 10 | 300 | 120 | | 8.0 | 3 | 350 | 120 | GSST | 8.0 | 1 | 250 | 120 |
|

Table 2. Optimized Structure for Five Colors: Red, Green, Blue, Yellow, and Purplea
Table 2. Optimized Structure for Five Colors: Red, Green, Blue, Yellow, and Purplea
Material | Red | Green | Blue | Purple | Yellow |
---|
Ag | 100 | 100 | 100 | 100 | 100 | | 148 | 225 | 200 | 180 | 240 | GSST | 10 | 10 | 10 | 10 | 10 | | 63 | 100 | 113 | 80 | 161 | Total thickness | 321 | 435 | 423 | 370 | 511 |
|
The optical spectra of a 10 nm GSST film annealed at different temperatures for the same duration are presented in Fig. 4(a). Below 320°C, the optical spectra exhibit consistent behavior, with the reflectance decreasing with wavelength while the transmittance increases rapidly, indicating that the GSST thin film remains in an amorphous state. Subsequently, as the temperature goes up, both reflective and transmissive spectra become flat, and their values decrease significantly, suggesting higher absorption in this coating, consistent with the expected outcome during the phase change of GSST according to the refractive index changes. Upon reaching 350°C, the spectra maintain their shape but shift, potentially due to vacancy ordering and a rougher surface caused by crystallization and high temperature.
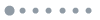
Figure 4.Properties of single-layer GSST films. (a) Spectra of samples with a thickness of 10 nm at different heating temperatures. (b) Spectra of samples with a thickness of 50 nm heated at 350°C for different heating time; (c) XRD patterns of films annealed at different temperatures. (d) Pictures of single-layer GSST films taken before and after phase transition.
Spectra obtained by heating 50 nm-thick GSST thin films at 350°C for various durations are presented in Fig. 4(b). The results indicate that the 50 nm single-layer GSST thin film begins to undergo a phase change at the fourth minute and fully converts to the c-state two minutes later. Notably, unlike the 10 nm samples, the effects of vacancy ordering and a rougher surface caused by high temperatures seem to diminish due to the relatively larger thickness. Furthermore, to validate these findings, an X-ray diffractometer is employed, and the measured data are illustrated in Fig. 4(c). Initially, owing to the high temperature of the electron beam and the high-energy bombardment of the ion beam, the films are in an amorphous state, with no visible diffraction peaks in the X-ray diffraction (XRD) patterns. After the annealing process, the GSST thin films crystallized, and diffraction peaks gradually formed at 20°, 48°, 55°, etc. The crystallization temperature is 320°C, well-consistent with the data from Fig. 4(a). In Fig. 4(d), images of single-layer GSST films before and after annealing are depicted. The first two samples are in an amorphous state with different thicknesses, both exhibiting a dark brown color with clearly visible text behind. However, as the film thickness increases, the text becomes increasingly blurred, suggesting lower transmission. After the annealing process, the sample acquires a metallic luster and becomes completely impermeable to light.
Five representative colors—red, green, blue, yellow, and purple—were carefully selected and fabricated to assess the potential application of GSST PCM in dynamically tunable structural colors. The layer arrangement for each color is documented in Table 2, maintaining a constant thickness of 10 nm for GSST thin films. This consistent thickness is chosen to enhance the color quality of the film stacks while maximizing their tunability. After the deposition process, the samples underwent annealing in a rapid thermal annealing (RTA) furnace to induce the transformation of GSST within the structure. In Figs. 5(b)–5(f), the measured spectra of these samples are displayed, where the blue line labeled “annealed,” the green dashed line labeled “design,” and the red dashed line labeled “as-deposited” represent the reflections at the stages of initial design, deposition, and annealing, respectively. The corresponding photographs are embedded in the images. Observations reveal that the peak reflectance of the five colors averages 92% in both GSST phase states, and after the transition, there is a 30% reduction in reflection at non-target wavelengths, resulting in vibrant c-state colors. The chromaticity diagram in Fig. 5(a) depicts the chromaticity coordinates of these colors before and after high-temperature stimulation. The blue solid signs represent “a” for amorphous, while the hollow signs represent “c” for crystalline. Typically, the distance from the edge reflects the quality of color performance; the closer to the edge, the better the saturation, indicating superior color performance; the coordinate variation between the two states signifies the tunability of the color, with a more pronounced change indicating a more tunable color; the size of the range covered by various colors represents the color gamut of the structure, namely the diversity of colors. Clearly, the coordinates of “c”-state samples are distinct from the “a”-state ones, signifying a different color appearance, especially for purple, green, and red colors. The color range covers the main gamut with center wavelengths ranging from 460 to 600 nm, enough for most application scenarios.
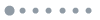
Figure 5.Optical spectra for five colors and their chromaticity coordinates: (a) chromaticity coordinates: solid for “a” representing amorphous and hollow for “c” representing crystalline; (b) red; (c) green; (d) blue; (e) yellow; and (f) purple (red for as-deposited, blue for annealed, and green for design).
While the simulation and experiments generally aligned well, several challenges were encountered during the experimental phase, notably film cracking and peeling, as depicted in Fig. 6(b). Films prepared using the ion-assisted electron beam evaporation method are typically flat and tight [as shown in Fig. 6(a)], and they exhibit clear demarcation. However, in this study, they developed cracks following the annealing process. The causes were attributed to stress mismatch induced by the stimulation process and imperfect deposition conditions. To address these issues, several related research avenues were explored: (a) adoption of materials with a similar coefficient of thermal expansion as connecting layers to buffer the stress caused by GSST’s high coefficient of thermal expansion, which can lead to crystal lattice distortion at elevated temperatures; (b) adjustment of the film deposition process, including the deposition rate and ion beam power, to match tensile and compressive stresses between different films; (c) optimization of the thermal annealing process parameters, such as temperature, annealing time, and RTA ramp rate; (d) recognition that GSST material is non-volatile. Once the stimulus intensity exceeds its threshold, GSST transforms from amorphous to crystalline and remains in this state even after cooling down. Faster stimuli, such as electricity and laser pulses, can mitigate the impact of the thermal effect. In Fig. 6(c), it presents a series of images obtained during the optimization of sample annealing time. The results suggest that the annealing time should not exceed 30 s to avoid ablating holes (as observed in the sample annealed for 5 min) and to minimize stress between different layers due to uneven temperature distribution.
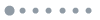
Figure 6.Experimental results. (a) SEM images (top left); freshly prepared physical samples (bottom left); control graphs of samples before and after annealing (right). (b) Film layer rupture (inset is the image after 10 times magnification under a microscope). (c) Process optimization of thermal annealing time.
3. Conclusion
In conclusion, a newly developed PCM, (GSST), has been successfully employed in the creation of tunable structural colors characterized by high color appearances and significant tunability. Historically, GSST material has predominantly found application in the infrared region within optoelectronic devices, with limited exploration in the visible region, thereby constraining its overall development. Here, we investigate its optical properties and potential application value at visible wavelengths, particularly in the realm of structural colors. In comparison to PCMs, GSST offers numerous advantages, including a large refractive index, low energy loss, and excellent optical contrast, which contribute significantly to enhancing the color quality of structures, including color gamut, saturation, and brightness, while still preserving high dynamic tunability. Distinct samples were successfully fabricated and transformed into colors—red, green, blue, yellow, and purple—utilizing a high temperature of 320°C as the stimulus. Undoubtedly, structural colors employing GSST material hold immense potential for various applications including non-volatile display devices, smart wearable technology, and so on. With further exploration and refinement, GSST-based structural colors are poised to revolutionize diverse sectors of technology and consumer electronics.