Light emitting diode (LED) applications in the fields of lighting and display have witnessed rapid growth alongside the progression of time and the heightening demands on its performance[1⇓-3]. CsPbBr3 quantum dots (QDs) were considered an ideal material for LED device fabrication due to their high photoluminescence (PL) intensity, photoluminescence quantum yields (PLQY) and color purity[4⇓-6]. However, the stability of colloidal CsPbBr3 QDs was relatively low, limiting their application in the fields of LED lighting and display. To address this issues, various strategies including ion doping[7⇓⇓-10], surface passivation[11-12], and encapsulation[13⇓⇓-16] were extensively explored by researchers to optimize and enhance the stability of CsPbBr3 QDs. Although these current approaches partially improved the stability of CsPbBr3 QDs, they could only mitigate external factors' corrosion on QDs, resulting in limited protection efficacy in practical applications.
Subsequently, the strategy of encapsulating CsPbBr3 QDs in glass to form glass-ceramic was discovered by researchers, which effectively isolated CsPbBr3 QDs from the external environment and significantly enhanced their stability[17⇓⇓⇓-21], which provided an ideal solution for improving the stability of CsPbBr3 QDs. However, the use of CsPbBr3 QDs coated with glass encapsulation, displays various challenges. Firstly, although glass is a transparent material, it inevitable scatters and refracts, attenuating the emitted light intensity and color purity of CsPbBr3 QDs. Secondly, due to its amorphous nature, the internal structure of glass is complex, necessitating precise manipulation to provide an optimal environment for the formation of CsPbBr3 QDs within the CsPbBr3 QDs@glass system. Furthermore, the composition of CsPbBr3 QDs also exerts an influence on the glass structure. An abundance of Cs promotes the formation of CsPbBr3 QDs within the glass matrix. However, an excessive amount of Cs+ disrupts the three-dimensional network structure of glass[22-23]. Another approach involves substituting PbBr with PbO[24], which significantly reduces volatility during solvation, enhances the luminescent intensity of CsPbBr3 QDs, and lowers production costs. Nonetheless, the higher toxicity of PbO than that of PbBr poses a challenge, and the redox implications during the melting process may cause color instability in the glass, thus detrimentally affecting the luminescent properties of CsPbBr3 QDs and hindering large-scale production of CsPbBr3 QDs@glass. Introducing Pb2+ as an extrinsic component into the glass matrix can partially alleviate its viscosity and optimize the glass structure. However, the large radius and high charge of Pb2+ exert a strong gravitational force on surrounding tetrahedral structures such as [SiO4] and [BO4][25], rendering the glass structure more densely packed, consequently impacting the luminescent intensity of CsPbBr3 QDs. Although there is still a need for further investigation into the influence of Pb2+ on the structure of CsPbBr3 QDs@glass, substantial findings regarding the overall impact of Pb2+ on glass have been accumulated[26⇓⇓-29]. Therefore, deeper understanding into the effect of Pb2+ on the structure of CsPbBr3 QDs@glass deserves further research.
Herein, CsPbBr3 QDs@glass were fabricated employing a melt-anneal approach. Delving into the thermal induction temperature, an in-depth exploration was conducted to investigate the influence of Pb2+ on the structural characteristics of CsPbBr3 QDs@glass, while refining and optimizing the PL intensity of CsPbBr3 QDs@glass. On this basis, the potential applications of CsPbBr3 QDs@glass in the domains of LED lighting and display were systematically explored.
1 Experimental
1.1 Chemicals
Silica (SiO2, 99.95%) was purchased from Xinyi Dahan Mining Co., Ltd. Boric acid (H3BO3, 99%) was purchased from Sinopharm Chemical Reagent Co., Ltd. Zinc oxide (ZnO, 99%), calcium fluoride (CaF2), cesium carbonate (Cs2CO3, 99%), lead bromide (PbBr2), sodium bromide (NaBr), lead oxide (PbO) were purchased from Aladdin Biochemical Technology Corp. All chemicals were used without any further purification.
1.2 Fabrication of CsPbBr3 QDs@glass
The glass composition consists of 85SiO2-170H3BO3- 55ZnO-5CaF2-16Cs2CO3-5PbBr2-15NaBr-0.5PbO. 40 g of the above raw materials were weighed and poured into a mixing tank for 2 h mixing at 25 Hz. The mixed raw materials were poured into the preheated platinum crucible, and then the lid was closed. Subsequently, all raw materials were melted in a high-temperature electric furnace at 1250 ℃ for 30 min. Afterward, the melted glass was poured into a preheated steel mold at 370 ℃ and then the molten glass was formed. Whereafter, the formed glass was returned to the muffle furnace at 370 ℃ for removal of stress. At this point, the precursor glass was obtained. Precursor glass was heat-treated by crystallization furnace in the range from 440 to 510 ℃ for 2 h. Finally, CsPbBr3 QDs@glass was obtained. The preparation steps were illustrated in Fig. 1.
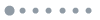
Figure 1.Schematic of preparation of quantum dots glass- ceramicsAC: After crystallization; BC: Before crystallization
1.3 Structural characterization
X-ray diffraction system (XRD, D/max 2550 V, RIGAKU, Japan) with Cu Kα radiation (λ=0.1542 nm) was used to detect the phase structure of CsPbBr3 crystals. Transmission electron microscopy (TEM, JEM-2100F, JEOL, Japan) with energy dispersive X-ray (EDX) was used to observe the microscopic morphology and size of a sample. Fourier transform infrared spectroscopy (FT-IR, EQUINOX55, Bruker, Germany) was used to test the structure of glass. X-ray photoelectron spectroscopy (XPS, Escalab250Xi, Thermo Fisher, USA) was used to test the components and change in non-bridging oxygen bonds. The samples used for testing were all in powder state.
1.4 Optical characterization
The PL spectra, and PLQY were recorded by a steady- state transient fluorescence spectrometer (PL, Fluorolog-3, HORIBA, Japan). The steady-state measurement system adopted a 450 W continuous Xenon lamp and CCD detector, and the transient measurement system adopted a new high-frequency pulse light source and a 980 nm pulse width continuous adjustable laser. When carrying out the spectrum test with an excitation wavelength of 365 nm, the integration time was selected as 5 s. Meanwhile, excitation light slit width was 3 nm, emission light slit width was 2 nm, and the test wavelength was in the range from 450 to 600 nm. All samples were powder state.
1.5 Fabrication and testing of LED device
The LED device was prepared by 2 g of polydimethylsiloxane (PDMS) with a molar ratio of CsPbBr3 QDs@glass powder, and n(powder) : n(PDMS) = 1 : 32. In order to mix evenly, the powder was magnetically stirred with PDMS for 2 h. Subsequently, the powder- glue mixture was dropped onto a 365 nm UV chip, and finally a green LED was obtained. The optical performance test of LED devices used a comprehensive optical performance characterization system (Varian, UK). During the test, a power supply with a power from 3 to 5 W was used.
2 Results and discussion
To investigate the influence of heat treatment on QDs microcrystalline glass and establish an optimal thermal processing protocol for subsequent research, this study initially obtained a pristine and transparent borosilicate glass precursor under the melting conditions of 1250 ℃ for 30 min. Subsequently, with thermal induction time of 2 h, heat-treatment was conducted on the glass precursor, and the results for thermal induction temperatures ranging from 440 to 510 ℃ are shown in Fig. 2.
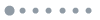
Figure 2.Crystal structures and optical properties of PQDs@glass at different temperatures for 2 h(a) XRD patterns; (b) PL spectra; (c) PLQY and PL intensity; (d) Fluorescence lifetime curvesColorful images are available on website
Fig. 2(a) exhibits the XRD patterns of CsPbBr3 QDs@glass thermal inducted in temperatures ranging from 440 to 510 ℃ for 2 h. The data in the graph are compared with PDF#54-0752, indicating successful synthesis of CsPbBr3 QDs@glass at different temperatures. Moreover, as the temperature is elevated, the crystalline peaks become more pronounced.
Subsequently, fluorescence spectroscopy was used to characterize the samples at different temperatures, and the results are shown in Fig. 2(b-d). Fig. 2(b) depicts the PL spectra, revealing a noticeable redshift in the emission center as the temperature increasing. To investigate the underlying cause, TEM characterization was conducted, and the size distributions of CsPbBr3 QDs at different temperatures were analyzed (Fig. 3(g-k)). The results demonstrate a gradual increase in the size of CsPbBr3 QDs with the rise of temperature. At 450 ℃, the QDs predominantly have a size distribution around 5 nm. In the temperature range from 460 to 490 ℃, over 86% of the particles fall within the size range of 6-14 nm. When the temperature reaches 510 ℃, the size distribution centers around 40 nm. This observed redshift in the emission center can be explained by the quantum size effect, where the bandgap of QDs decreases as their size increases (Fig. 3(f)).
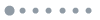
Figure 3.TEM characterization and size distribution analyses of PQDs@glass(a, b) TEM images before (a) and after (b) heat-treatment; (c) High-resolution TEM image; (d, e) TEM image and EDS mappings; (f) Schematic diagram of the relationship between size and band gap of CsPbBr3 QDs; (g-k) TEM images and size statistics
Furthermore, the TEM images clearly indicate that the quantity of QDs increases within the temperature range from 450 to 490 ℃. Initially, during the nucleation stage, the increased number of QDs leads to enhance PL intensity and PLQY. However, as the number of QDs continues to increase, self-absorption becomes more pronounced, resulting in a decrease in PL intensity and PLQY (Fig. 2(c)). Additionally, the fluorescence spectra demonstrate that the highest PL intensity is achieved at 460 ℃, while the PLQY reaches a peak value of 95.6% at 450 ℃.
What follows are a further exploration of the microstructure of CsPbBr3 QDs@glass, as shown in Fig. 3(a-e). Additional evidence of the successful synthesis of CsPbBr3 QDs within the borosilicate glass matrix is provided by Fig. 2(a-c). Particularly, the high-resolution TEM image (Fig. 3(c)) reveals an interlayer spacing of 0.26 nm, corresponding to the (210) crystal plane of CsPbBr3 QDs. The mapping images in Fig. 3(d, e) illustrate the accumulation of Cs+, Pb2+, and Br- ions within the region where the QDs precipitate. This observation aligns with previous studies suggesting that the abundance of Cs+ contributes to the formation of CsPbBr3 QDs.
With the goal of enhancing luminescence intensity, this study further investigated the influence of Pb2+ ions on the structure of CsPbBr3 QDs@glass, building upon the aforementioned research. The regulation of Pb2+ ions composition is presented in Table 1.

Table 1.
Component regulation of Pb2+ ions (all data in molar ratios)
Table 1.
Component regulation of Pb2+ ions (all data in molar ratios)
Code | SiO2 | H3BO3 | ZnO | CaF2 | Cs2CO3 | PbBr2 | NaBr | PbO |
---|
Pb-1 | 85 | 170 | 55 | 5 | 16 | 4 | 17 | 0.5 | Pb-2 | 85 | 170 | 55 | 5 | 16 | 5 | 15 | 0.5 | Pb-3 | 85 | 170 | 55 | 5 | 16 | 6 | 13 | 0.5 | Pb-4 | 85 | 170 | 55 | 5 | 16 | 7 | 11 | 0.5 |
|
This resulted in a tighter glass structure and increased density. Pb2+ ions were able to form more bond connections within the glass network, which contributed to strengthening the three-dimensional network structure of the glass. Due to the higher charge and larger ion radius of Pb2+, it exerts a strong attractive force on the surrounding [SiO4] and [BO4], stabilizing the entire network structure. The decreased transparency from Pb-1 to Pb-4 further supported this observation. As a direct consequence, the glass precursor of Pb-1 formed a looser structure, facilitating the migration of Cs+, Pb2+, and Br- ions during annealing and stress relaxation processes, thus resulting in crystallization. With the increasing concentration of Pb2+ ions, the density of the glass gradually increased. However, when it exceeded a certain range, it led to the formation of more interfaces within the precursor. These interfaces lowered the energy barrier for the formation of nanocrystals by Cs+, Pb2+, and Br- ions. Therefore, a small portion of crystals reappeared in the precursors of Pb-3 and Pb-4.
Fig. 4(a) presents the glass precursors prepared with different conditions of Pb2+ ions. The results indicate that extensive crystallization did not occur in Pb-2, Pb-3, and Pb-4 precursors, whereas Pb-1 precursor exhibits crystal formation due to the larger radius of Pb2+ ions that occupied more space in the lattice structure and increased the atomic packing density of the glass.
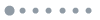
Figure 4.(a) Photographs, (b) XRD patterns and (c) PL spectra of CsPbBr3 QDs@glass with different Pb2+ concentrations
The XRD results after thermal induction at 460 ℃ for 2 h (Fig. 4(b)) aligned with the precursor results (Fig. 4(a)). After thermal induction treatment, Pb-1 displays prominent diffraction peaks, while Pb-2, Pb-3, and Pb-4 do not. The obvious diffraction peaks in Pb-1 can be attributed to the substantial crystallization that have already occurred in its precursor (as depicted in Fig. 4(a)). Consequently, an increased number of crystals are formed during the thermal induction process, thereby resulting in the distinct diffraction peaks.
At this stage, the luminescent performance of the samples was characterized, and it was observed that Pb-3 exhibited the optimal results. This indicates that an inadequate concentration of Pb2+ in the precursor leads to the occurrence of uncontrollable crystallization, resulting in a reduction in the final PL intensity. Likewise, an excessive concentration of Pb2+ results in a high density of the borosilicate glass precursor, hindering the migration of Cs+, Pb2+, and Br- ions necessary for the formation of CsPbBr3 QDs. Consequently, this excessive density contributes to a decrease in PL intensity.
To investigate the impact of Pb2+ ions on the luminescent properties of CsPbBr3 QDs in glass, we further employed FT-IR and XPS to delve into the glass structure. The results are shown in Fig. 5(a-c). Fig. 5(a) illustrates that the glass network structure is primarily composed of polyhedra such as [BO3], [BO4] and [SiO4]. The peak at 710 cm-1 corresponds to the bending vibration of B-O bonds in the [BO3] groups. The broad peak at 1024 cm-1 corresponds to the stretching vibrations of both [BO4] groups and Si-O-Si bonds. The shoulder peak at 1272 cm-1 corresponds to the vibration of [BO3] polyhedra, while the shoulder peak at 1375 cm-1 corresponds to the asymmetric stretching vibration of [BO3] polyhedra.
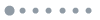
Figure 5.Structural characterization of PQDs@glass at different Pb2+ concentrations(a, b) FT-IR and XPS spectra, and (c) the ratio of bridging to non-bridging oxygen bonds; Colorful figures are available on website
From Pb-1 to Pb-4, a broad peak at 1024 cm-1 noticeably shifts towards higher wavenumber, indicating an increasing contents of [SiO4] and [BO4]. This suggests that the glass network structure become more compact with the increasing concentration of Pb2+. It aligns well with our previous speculation regarding the occurrence of uncontrolled crystallization in the precursor. Moreover, through XPS analysis, we investigated the ratio of bridging oxygen bonds to non-bridging oxygen bonds in the borosilicate glass (Fig. 5(c)). The results indicate that the proportion of bridging oxygen bonds gradually increases as the Pb2+ concentration rises. This explains the aforementioned hypothesis that “Pb2+ exerts a stronger attraction on neighboring [SiO4] and [BO4], contributing to the enhancement of the three-dimensional network structure of the glass.”
Subsequently, this study evaluated the water resistance, UV irradiation resistance, and thermal cycling stability of the samples. The results indicate that the PL intensity can maintain over 70% of its initial value even after immersion for 360 h (Fig. 6(a)). Similarly, the PL intensity remains above 70% of its initial value after exposure to UV radiation for 210 h (Fig. 6(b)). The primary reasons for the attenuation of its water stability and UV stability are mainly due to the fact that, during the high-energy ball milling process, the powder material develops numerous cracks, resulting in a greater number of QDs being exposed. Consequently, these exposed QDs are more susceptible to environmental influences, which lead to a significant decrease in their intensity. And after undergoing 10 cycles of thermal cycling, the luminescence intensity of CsPbBr3 QDs@glass retains 98.9% of its initial value without significant decrease. The luminescence intensity for each individual cycle maintains over 80% of its initial value. Moreover, the full width at half maxima (FWHM) is relatively stable at around 24 nm, without significant change (Fig. 6(c)).
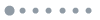
Figure 6.Stability experiments of PQDs@glass(a, b) Variations in PL intensity and FWHM of water resistance (a) and UV stability (b), and (c) experimental results of thermal cycling stability
Finally, the material was mixed with PDMS and combined with a 365 nm UV chip to create LED microspheres. The characterization results (Fig. 7(a)) show that the LED samples produced using Pb-2 as the base material after thermal induction at 460 ℃ for 2 h have an emission center at 517 nm (Fig. 2(b)), closely matching the original material’s emission center at 518 nm. Additionally, Fig. 7(b) reveals that the LED color coordinates achieved with Pb-2, Pb-3, and Pb-4 are (0.21, 0.62), (0.16, 0.60) and (0.14, 0.53) respectively, encompassing a spectrum of 110% sRGB color gamut and extending the boundaries of color space.
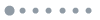
Figure 7.(a) LED device fabricated with CsPbBr3 QDs@glass, and (b) CIE coordinates of CsPbBr3 QDs@glass LED devices at different Pb2+ concentrations
3 Conclusions
In this study, we successfully prepared high-luminescence PQDs@glass under the conditions of a thermal induction temperature of 460 ℃ and a Pb2+ concentration of 6 mol. Based on this, we systematically investigated the influence of Pb2+ ions on the structure of PQDs@glass, and found that an increase in Pb2+ content leads to a densification of the microstructure of PQDs@glass, thereby affecting the diffusion of glass components and resulting in a change in the luminescence intensity of PQDs@glass. Subsequently, LED devices prepared from these materials expanded the standard RGB color gamut. This study extends the potential applications of CsPbBr3 QDs@glass in the fields of LED lighting and display, laying the foundation for the large-scale preparation of CsPbBr3 QDs@glass.