Introduction
The applications such as machine learning and cloud computing demand advanced computing systems with high computational power and high power efficiency. The cryogenic computing such as single-flux-quantum (SFQ) superconducting computing and quantum computing with high speed and low power consumption is a promising computing technology[1, 2]. Since the SFQ superconducting circuits operate at 4 K environment, it is challenging to establish a high-speed and energy-efficient data link between the cryogenic temperature and the room temperature (RT). Optical interconnect based on the cryogenic vertical-cavity surface-emitting laser (VCSEL) is considered as a promising solution due to the low power consumption, high speed, and low thermal leakage, compared to conventional electrical interconnect[3].
Cryogenic VCSELs are ideal laser sources for the transmitters operating at cryogenic temperature, since cryogenic VCSELs have high power efficiency, high modulation bandwidth, circular beam for a high coupling efficiency with a multi-mode fiber, and small footprint. The first cryogenic VCSEL with a 16-μm proton-implant aperture was demonstrated in 1996[4]. This cryogenic VCSEL had a threshold current of about 2.8 mA at 6 K and showed a −3-dB modulation bandwidth of 11 GHz. A cryogenic oxide-confined VCSEL at 145 K achieved 10 Gbps data rate with an average energy dissipation of 180 fJ/bit in 2012[5]. Then efforts have been made to enhance the modulation bandwidth and the energy efficiency of the cryogenic oxide-confined VCSEL at cryogenic temperature. The cryogenic oxide-confined VCSEL realized a threshold current of 0.2 mA at 7.5 K, and 50 GHz small-signal bandwidth at 82.1 K in 2020[6]. Driven by a superconducting bit pattern generator with a peak-to-peak voltage (Vpp) of 250 mV, the cryogenic oxide-confined VCSEL at 4 K achieved an error-free data rate of 12.5 Gb/s at 1.8 mA in the non-return-to-zero format with an energy efficiency of 371.3 fJ/bit[7]. At 2.8 K, the cryogenic oxide-confined VCSEL with a threshold current of 0.2 mA realized a −3-dB bandwidth of larger than 50 GHz and 128 Gbps PAM-4 data rate at 7 mA, which shows a potential energy efficiency of sub-100 fJ/bit at cryogenic temperature[8].
In this paper, we demonstrate a cryogenic 850-nm oxide-confined VCSEL, targeting the application in the cryogenic optical interconnect. The cryogenic VCSEL with an optical oxide aperture of 6.5 μm in diameter can operate in single fundamental mode with a side-mode suppression-ratio (SMSR) of 36 dB at 3.6 K. The small signal responses of the fabricated VCSEL measured at RT and 292 K show the potential to achieve high dynamic performance at cryogenic temperature.
Design and fabrication
Fig. 1(a) shows the cross-sectional schematic of the cryogenic VCSEL structure, which is developed from the VCSEL operating at RT[9]. The epi-structure of the VCSEL is composed of a 34-pair bottom Al0.12Ga0.88As/Al0.9Ga0.1As distributed Bragg reflector (DBR), a 0.5λ-cavity with multiple strained InGaAs quantum wells (QWs), and a 25.5-pair top Al0.12Ga0.88As/Al0.9Ga0.1As DBR. Two Al0.98Ga0.02As layers and four Al0.96Ga0.04As layers above the active region in the top DBR are employed to reduce the parasitic effect. Graded interfaces and modulation doping schemes are used for the DBR to reduce the loss and the resistance.
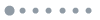
Figure 1.(Color online) (a) Cross-sectional schematic of the cryogenic VCSEL structure. (b) Packaged cryogenic VCSEL mounted in a cryostat.
The gain peak wavelength of the QWs shifts faster (~0.3 nm/K) than the cavity mode wavelength (~0.06 nm/K) when the temperature changes[5, 10]. In the cryogenic VCSEL design, the cavity mode (F−P dip) is deliberately placed at the shorter wavelength range of the gain peak (approximated by the photoluminescence (PL) peak) of the InGaAs QWs at RT to realize a high performance of the VCSEL at cryogenic temperature, as schematically shown in Fig. 2. When the temperature decreases, the cavity mode can be aligned well with the gain peak at 4 K, and the VCSEL has a low threshold current. Therefore, the wavelength detuning between the gain peak and the cavity mode affects the performance of the VCSEL at both RT and cryogenic temperature.
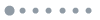
Figure 2.(Color online) Schematic of the temperature-dependent misalignment between the gain peak and the F−P dip.
The cryogenic VCSELs are fabricated with the processing flow of high-speed oxide-confined VCSELs[9]. The p-contact is deposited by the lift-off process. A mesa is formed by etching a ring air trench to expose the Al0.98Ga0.02As layers, and followed by lateral oxidation process to form the oxide apertures. The whole VCSEL epitaxial layers are etched down to the n-contact layer, and then the n-contact is deposited. The ground-signal (GS) coplanar pads are deposited on top of the contact layer and above the benzocyclobutene (BCB) islands to reduce parasitic capacitance.
Experimental results
The cryogenic VCSEL is packaged with an OM3 multi-mode fiber for the measurement in a cryostat, as shown in Fig. 1(b). The temperature is monitored by a Lakeshore temperature controller. The direct current (DC) to the VCSEL is supplied by a souremeter (Keithley 2400). Fig. 3(a) shows the fiber-coupled output power−current−voltage (L−I−V) curve of the cryogenic VCSEL at RT (298 K). Since the VCSEL is designed for the operation at cryogenic temperature, the cavity mode wavelength is deliberately blue-shifted away from the PL peak of the QWs at RT. The cavity mode wavelength is located at the edge of the gain spectrum at RT. Therefore, the threshold current of the VCSEL at RT is as high as 5 mA and the thermal rollover occurs at 6.1 mA, because of the low modal gain and self-heating effect.
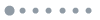
Figure 3.(Color online) (a) Measured L−I−V curves of the cryogenic VCSEL at RT (298 K). (b) Measured L−I−V curve of the cryogenic VCSEL at 3.6 K. (c) Lasing spectra of the cryogenic VCSEL at 5 mA within the temperature range from 298 to 3.6 K. (d) Lasing spectra of the cryogenic VCSEL under different currents at 3.6 K. (e) Wavelengths of the fundamental mode of the cryogenic VCSEL at 5 mA under different temperatures. (f) Wavelengths of the fundamental mode of the cryogenic VCSEL at 3.6 K under different currents.
When the temperature decreases to 3.6 K, as shown in Fig. 3(b), the threshold current of the VCSEL is 1.5 mA and the fiber-coupled output power is 1.7 mW at 8 mA, due to the small wavelength detuning between the gain peak and the cavity mode at 3.6 K. However, the voltage of the VCSEL at 3.6 K is 5.28 V at 6 mA, two times of that (2.61 V at 6 mA) at RT, because of the reduced thermionic emission and thermally assisted tunneling through the barrier at cryogenic temperature[11]. We also find that, the differential resistance of the VCSEL is 57 Ω under 6 mA at RT, whereas the differential resistance is 1.5 Ω under 6 mA at 3.6 K. The low differential resistance at 3.6 K is due to the low doping concentration[10]. Increasing the doping concentration can increase the differential resistance of the cryogenic VCSEL to 50 Ω, beneficial to the impedance matching for the dynamic performance.
The spectra of the cryogenic VCSEL at a bias current of 5 mA under different temperatures are measured with an optical spectrum analyzer AQ6317, as shown in Fig. 3(c). The size of the optical oxide aperture of the cryogenic VCSEL is 6.5 μm in diameter at 298 K, calculated from the mode spacing between the fundamental mode and the first higher-order mode[9]. The VCSEL operates in multiple modes at 5 mA at RT. As the temperature decreases, the VCSEL operates in multiple modes till 6 K. However, at 3.6 K the VCSEL operates in single fundamental mode with a SMSR of 36 dB and has a fiber-coupled output power of 1 mW at 5 mA, even though the VCSEL has an optical oxide aperture of 6.5 μm in diameter. The reason for the single-fundamental-mode operation of the VCSEL at 3.6 K may be as follows. The oxidized region in the VCSEL has a lower effective thermo-optic coefficient change than that of the non-oxidized region when the temperature decreases from 298 to 3.6 K. Thus, the effective refractive index change of the oxidized region in the VCSEL is smaller than that of the non-oxidized region when the temperature decreases from 298 to 3.6 K. Then the optical confinement introduced by the oxide layer in the VCSEL at 3.6 K is weaker than that at 298 K. The higher-order modes have higher losses and the fundamental mode is preferred to lase. To the best of our knowledge, it is the first time to demonstrate the single-fundamental-mode (SMSR > 30 dB) VCSEL with an oxide aperture of about 6 μm at around 4 K[5, 12, 13].
As shown in Fig. 3(c), the full width at half maximum (FWHM) of the fundamental mode of the VCSEL at 298 K is 0.077 nm. As the temperature decreases to 130 and 3.6 K, the FWHM of the fundamental mode are 0.059 and 0.037 nm, respectively. This indicates that the quality factor of the optical cavity of the VCSEL is increased at 3.6 K compared with that at RT.
We further measure the spectra of the VCSEL at 3.6 K at different currents, as shown in Fig. 3(d). The device operates in single fundamental mode till 5 mA with a SMSR of large than 30 dB. At 5.5 mA and beyond the device operates in multiple modes. These results indicate that, the self-heating can affect the modal losses of the higher-order modes of the VCSEL at 3.6 K, and the higher-order modes have higher losses and are suppressed at 3.6 K.
From 298 to 3.6 K, the fundamental mode of the VCSEL shifts from 863.11 nm (5 mA) at RT to 850.31 nm at 1.5 mA near the threshold current at 3.6 K, as shown in Fig. 3(e). As the temperature decreases, the wavelength-temperature coefficient dλ/dT changes nonlinearly, from 0.056 nm/K at 210 K to 0.011 nm/K at 3.6 K. The nonlinear shifting of the fundamental mode of the VCSEL is due to that the thermo-optic coefficients of the AlGaAs-based materials and the oxide layer decrease nonlinearly as the temperature decreases[14−16]. At 3.6 K, the thermo-optic coefficient is much lower than that at RT. The cavity length of the VCSEL is almost constant when the temperature approaches 4 K, and the wavelength shifts slowly at around 4 K. Fig. 3(f) shows the wavelengths of the fundamental mode of the cryogenic VCSEL at 3.6 K under different currents. For our VCSEL with an optical oxide aperture of 6.5 μm in diameter, as the current increases the wavelength of the fundamental mode increases nonlinearly with coefficients dλ/dI of 0.19 nm/mA at 2 mA to 0.44 nm/mA at 6 mA. Even though the ambient temperature is 3.6 K, the self-heating due to the parasitic resistance of the VCSEL with an injected current can increase the junction temperature.
The VCSEL is intentionally designed to operate at cryogenic temperature with high performance. Because currently there is no available cryogenic on-wafer probe station with a high-frequency microwave probe and a fiber holder, we have not yet obtained the ideal dynamic characteristics of the VCSEL at cryogenic temperature. However, we perform the small signal modulation response S21 measurements of an identical VCSEL at 298 and 292 K in the open circumstance. The VCSEL chip is mounted on a carrier with ground-signal-ground (GSG) pads, and placed on a holder connected to a thermoelectric cooler (TEC) controller. The small signal from a Keysight network analyzer (N5247B) and the DC from a souremeter (Keithley 2401) are combined by a bias tee (SHF BT65R-D) and injected to the VCSEL through a microwave probe. The output power of the VCSEL is connected by an OM3 fiber and coupled to a photoreceiver (Thorlabs RXM25BF). Fig. 4 shows the measured small signal response S21 curves of the VCSEL at RT and 292 K. Note that, the small signal response S21 includes the photoreceiver response and the probe response. At RT, the modulation bandwidth f−3dB is 3.9 GHz at 6 mA. However, when the temperature decreases to 292 K, the modulation bandwidth f−3dB is 6.9 mA under a bias current of 7 mA.
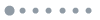
Figure 4.(Color online) (a) Small signal responses S21 of the VCSEL at RT (298 K). The inset shows the VCSEL chip mounted on a carrier with GSG pads. (b) Small signal responses S21 of the VCSEL at 292 K.
The fabricated VCSEL here is not as good as a typical high-speed VCSEL operating at RT, because the cavity mode is placed far away from the gain peak at RT, as shown in Fig. 2. However, as the temperature decreases, the detuning between the gain peak and the cavity mode decreases. Therefore, the threshold current will decrease, and the output power will increase. The relaxation resonance frequency of the VCSEL is proportional to the square root of the photon density in the cavity[17]. These small signal response results in Fig. 4 and the power−current curves in Figs. 3(a) and 3(b) indicate that, the fabricated VCSEL has the potential to achieve a higher modulation bandwidth when the temperature decreases to 4 K. In future, we will study the dynamic characteristics of the VCSEL at cryogenic temperature with an improved high-frequency package.
Conclusion
We demonstrate a cryogenic 850-nm oxide-confined VCSEL. At 3.6 K the VCSEL operates in single fundamental mode with a SMSR of 36 dB and has a fiber-coupled output power of 1 mW at 5 mA. The cryogenic VCSEL is very promising for the cryogenic computing which needs high-speed and energy-efficient data links between the cryogenic temperature and the RT. In future, the dynamic performance and energy efficiency of the cryogenic 850-nm oxide-confined VCSEL will be studied at 4 K, and the threshold current will be reduced for a high energy efficiency.