1. Introduction
Since the discovery of graphene, a variety of two-dimensional layered van der Waals (vdW) materials have been synthesized and their unique and attractive physical properties have been reported[1−3]. Due to the absence of suspension keys on the surface, van der Waals heterojunctions do not require strict lattice matching, so they can be combined flexibly and conveniently, opening up more possibilities for transistor performance optimization and function development. Conventional heterojunction transistors have diode rectification characteristics that allow only a single direction of current to pass between the heterojunction regions. At present, the more popular bidirectional rectifier diode is composed of two pn junction diodes, when a voltage is applied to one of the pn junctions, the junction is in the conduction state, and the other is in the cut-off state, and vice versa, so that the current can be turned on in both positive and negative directions, to achieve the effect of bidirectional rectification. Therefore, bidirectional rectifier diodes are extensively applied to the control of reversible DC motors, converters, inverters, and other fields[4]. Compared with unidirectional rectifier diodes, bidirectional rectifier diodes have better efficiency, faster response speed, and a wider range of application scenarios. However, due to the need for two diode combinations, it is difficult to break through the size reduction of large-scale integration. Therefore, researching the bidirectional rectification characteristics of individual heterojunctions will pave the way for the size reduction of integrated circuits. As a bipolar semiconductor material[5], WSe2 can be adjusted by gate voltage to control its carrier concentration and doping amount, to exhibit different polarities[6, 7]. At present, the bidirectional rectification behavior of WSe2-based heterojunction has arisen[8−10]. In the WSe2/GeSe heterojunction, the WSe2 Fermi level can be regulated over the entire bandgap range by adjusting the external gate voltage, and bidirectional rectification is implemented[11]. Generally, the reverse leakage current increases due to the minority carriers during reverse bias, resulting in a low rectification ratio[12]. However, the type Ⅱ band structure can suppress the leakage current through the interface barrier, to achieve a high rectification ratio[13]. Bi2O2Se material not only forms a type Ⅱ band alignment with WSe2 but also has attracted extensive attention because of its ultra-high mobility. In the Bi2O2Se and WSe2 heterojunctions, the carriers on the side of the Bi2O2Se with high mobility are cycled several times to generate gain, thereby improving the photoelectric responsivity. At the same time, as long as the Bi2O2Se/WSe2 junction is very sharp and there is no additional oxide layer, the photoelectric response speed can rise substantially.
In this work, we fabricated a WSe2/Bi2O2Se multilayer vdW heterojunction transistor on a silicon substrate by manual stacking. Fig. 1(a) shows a schematic diagram of the atomic arrangement of the Bi2O2Se/WSe2 FET, with the white frame line pointing to the arrangement of the atoms in the heterojunction region. WSe2 is a typical sandwich structure, with the layers connected by van der Waals forces. The bottom Bi2O2Se is alternately distributed in the [Se]n2n− layer and [Bi2O2]n2n+ layer, and the WSe2 and Bi2O2Se are also combined by weak van der Waals forces. Bi2O2Se has a non-electrically neutral layered structure[14]. The electric field that may exist around the material promotes electron−hole separation when the junction is formed, similar to the built-in electric field. The Bi2O2Se/WSe2 heterojunction transistor we prepared not only has the basic rectification characteristics of diodes, but also can realize the reverse flow of current in the heterojunction region under the control of gate voltage, and has bidirectional rectification characteristics. The rectification ratio (If/Ir) approaching 105. The current on/off ratio of the WSe2/Bi2O2Se transistor can be changed by nearly five orders of magnitude under the control of the gate voltage, with the current on/off ratio (Ion/Ioff) exceeding 105. The current on/off ratio is expected to be further improved under the modulation of gate voltage. The photocurrent characterization of the WSe2/Bi2O2Se heterostructure verifies the bidirectional switching function of the device. Ultraviolet photoelectron spectroscopy (UPS) proved that the band shift belonged to type Ⅱ, and the difference in the work function of the two semiconductor materials under Kelvin probe force microscopy (KPFM) was completely consistent with the UPS results. We combine the photocurrent test results with the band structure to explain the mechanism of bidirectional regulation of photocurrent by gate−source voltage in heterojunction. In addition, visible light has an excellent spectral response to the near-infrared region, with a maximum photocurrent value of 176 μA and a response time as low as microseconds.
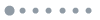
Figure 1.(Color online) (a) Schematic diagram of WSe2/Bi2O2Se FET. (b) Optical micrograph of the WSe2/Bi2O2Se FET.
2. Method
Device fabrication The Bi2O2Se/WSe2 heterojunction was prepared by the aligned dry transfer method. Tilt-grown Bi2O2Se (30 nm) prepared by chemical vapor deposition on mica substrate is transferred to a clean SiO2/Si substrate by direct compression[15]. Multilayers of WSe2 (40 nm) were directly stripped from WSe2 bulk materials (2D Semiconductors, China) using mechanical stripping and transferred to polydimethylsiloxane (PDMS) substrates. Observe under the microscope to find suitable WSe2 regions and Bi2O2Se for physical marks. Under a 2D transfer system, the PDMS substrate is inverted above the transfer region and the SiO2/Si substrate with Bi2O2Se is placed below the transfer region. Using a microscope to align the physically labeled area precisely, slowly descend WSe2 until it comes into contact with Bi2O2Se, and slowly raise the PDMS after heating at 80 °C for 10 min, the transfer of the 2D material is completed. Because of the thermal release of polydimethylsiloxane, heating can attenuate the viscosity between WSe2 and PDMS, and the WSe2 and PDMS are separated after heating due to the van der Waals force between the two 2D materials. We choose the high-function Pd as the contact material to form a larger Schottky junction, thereby reducing the dark current. Metal Pd film is soft and difficult to be transferred by probe under microscope, so we steamed a layer of Au film on the surface of Pd film to facilitate electrode transfer. The Pd/Au electrodes were prepared by vacuum evaporation, the Pd/Au films with thicknesses of 10 nm/100 nm were evaporated sequentially on the SiO2/Si substrate, and the copper TEM grid was used as a mask to obtain strip Pd/Au electrodes arranged in parallel on the SiO2/Si substrate. We used probes to transfer the electrodes and build them on either side of the Bi2O2Se/WSe2 heterojunction. The Bi2O2Se/WSe2 heterojunction FETs were annealed for 1 h at a vacuum of 8 Pa, 200 °C, and without a carrier gas. The Bi2O2Se/WSe2 heterojunction optical image is illustrated in Fig. 1(b), with the red border area being WSe2, the green dotted box being Bi2O2Se, and the blue dotted area being the Pd electrode.
Device characterization Device electrical curves were obtained using Keysight B1500 SMUs. The surface potential of the sample was measured with an atomic force microscope (AFM, Park) before electrode attachment. The ultraviolet photoelectron spectra of Bi2O2Se and WSe2 prepared under the same experimental conditions were collected by UPS (Thermoscalab250X). The photoresponse experiment was performed under 640 nm laser irradiation using a laser spot with a diameter of 1 cm. The response velocity of the Bi2O2Se/WSe2 heterojunction transistor was measured using a data acquisition card (NI PCIe-6321). The identical sample was used for both the optoelectronic and AFM tests of the device.
3. Results and discussion
After the device was fabricated, we tested the electrical performance of the device. Unlike common heterogeneous transistors with diode rectification characteristics[16], Figs. 2(a) and 2(b) show the output curves at gate voltages of 40 V and −80 V, respectively, with an inset of logarithmic coordinates. The Bi2O2Se/WSe2 heterojunction transistor we prepared exhibits bidirectional rectification under the control of gate voltage. We speculate that when the gate voltage is changed, the concentration of carriers in the semiconductor material changes accordingly through electrostatic doping, that is, the direction of the built-in electric field formed by the two semiconductor materials is reversed under the action of the gate voltage, so the rectification direction of the heterojunction device is observed. As shown in Figs. 2(c) and 2(d), the logarithmic plot of the transfer curve shows an equally spaced change in gate voltage from −80 to 40 V. When the source−drain voltage Vds > 0 V, the gate voltage Vg is positive, and the heterojunction device is in a conduction state. Conversely, when the source−drain voltage Vds < 0 V and the gate voltage Vg is positive, the heterojunction device is in a shutdown state, consistent with our previous measurement output curve. Figs. 2(e) and 2(f) show the logarithmic coordinates of the output curve at different gate voltages from −80 to 40 V, showing the forward and reverse rectification, respectively. It can be discovered that between the gate voltage of −40 and −30 V, the direction of rectification changes due to the displacement of the Fermi level in the semiconductor material WSe2 by electrostatic doping.
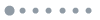
Figure 2.(Color online) (a) and (b) Output curves at gate voltages of 40 and 80 V with an inset of logarithmic coordinates. (c) and (d) Logarithmic diagram of the transfer curve at different bias voltage Vds. (e) and (f) Current liner diagram in logarithmic coordinates, recorded by scanning gate voltage Vg.
Fig. 3(a) shows the 3D image of the source−drain voltage from −5 to 5 V and the gate voltage from −80 to 40 V. As can be seen from the figure, the source leakage current is controlled by both the gate voltage and the source leakage voltage, and the Bi2O2Se/WSe2 heterojunction transistor exhibits different conductivity due to the change of gate voltage. Fig. 3(b) shows current on/off ratios for the device at source−drain voltages of ±100 mV, ±1 V, and ±3 V, Bi2O2Se/WSe2 heterojunction FET current on/off ratio of >105 at Vg = −80 V, even at a source−drain voltage of ±100 mV, the transistor's current on/off ratio is still greater than 102. As shown in Fig. 3(c), the transistor's rectification ratio reaches 105 at a gate voltage of −80 V and is greater than 10 even at forward bias. If we want to make the circuit conductive in both opposite directions, the simple logic circuit needs to control both transistors at the same time, because conventional diodes can only be single-conductive. But the difference is that we can take advantage of the Bi2O2Se/WSe2 heterojunction bidirectional rectification properties and directly use a transistor to control the direction of the current. Diode can be used to achieve triode function, which is expected to be applied to the size reduction of integrated circuits.
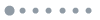
Figure 3.(Color online) (a) 3D-map of current, recorded by scanning gate voltage Vg and bias voltage Vds. (b) The rectifying ratio of the forward current If to the reverse current Ir at the same gate voltage. (c) On/off ration of the Bi2O2Se/WSe2 heterojunction.
The charge transport on the heterostructure is essentially governed by the bands, while the Fermi level is affected by electrostatic doping. We preliminarily determined the relative Fermi level positions between the two semiconductor materials using Kelvin probe force microscopy (KPFM). According to the formula [17] (where and is the work function of Bi2O2Se and WSe2, q is the charge of the electron, V is the surface potential of WSe2 and Bi2O2Se). As shown in Fig. 4(a), the difference in work function between the two semiconductor materials is about 127 meV. To obtain a more specific band alignment of the Bi2O2Se/WSe2 heterostructure, ultraviolet electron spectroscopy (UPS) was used to test, as shown in Fig. 4(b). The work functions (W) of Bi2O2Se and WSe2 are calculated to be 4.70 and 4.83 eV, respectively, by subtracting the second electron cut-off energy from the energy of the He I light source (21.21 eV), and the work functions of the two materials are consistent with the test results of KPFM. The distance between the edge of their valence band and the Fermi level is 0.66 and 0.44 eV, respectively. According to the multilayer Bi2O2Se with a bandgap of 1.2 eV, its electronic affinity is about 4.07 eV[18, 19]. Similarly, for bulk WSe2, the band gap and electron affinity are about 0.8 and 4.56 eV[20, 21], respectively. The conduction band edge and valence band edge of WSe2 are 0.49 and 0.09 higher than those of Bi2O2Se, respectively. Therefore, the band arrangement before and after the contact between Bi2O2Se and WSe2 is shown in Fig. 4(c), after the two materials come into contact, electrons flow to the WSe2 side with a higher work function, and the band bends under the action of the built-in electric field, as shown in the figure on the right.
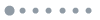
Figure 4.(Color online) (a) Kelvin Probe Force Microscopy (KPFM) image of the Bi2O2Se/WSe2 FET, the inset shows KPFM line scan, denoted by a white dotted line in figure. (b) Ultraviolet photoelectron spectra of WSe2 and Bi2O2Se. (c) Band diagrams of the Bi2O2Se/WSe2 heterojunction.
An unambiguous analysis of the effect of gate voltage on heterojunction devices requires an understanding of band structure variation. The application of gate voltage creates a vertical external electric field on the surface of the semiconductor material, and there is a significant electrostatic potential in the semiconductor. For electrons, a decrease in the electric potential along the direction of the electric field means that the potential energy increases, the energy level of the electron increases, and the energy band bends accordingly. Therefore, we tried to characterize the electrostatic potential distribution in semiconductors by photocurrent mapping to find the correlation. Carrier concentration in the raw material is estimated according to , where electron charge , the capacitance of 275 nm SiO2, the vacuum dielectric constant , the relative dielectric constant of 275-nm SiO2 3.9[22]. Supplement Fig. S1 shows the threshold voltages Vth of −25.13 and −32.46 V for WSe2 and Bi2O2Se, respectively. Then the carrier concentrations of WSe2 and Bi2O2Se are and , respectively. Lower carrier concentration of WSe2 than Bi2O2Se, coupled with direct contact between WSe2 and the substrate indicate that the influence of WSe2 Fermi energy level is more obvious in the process of electrostatic doping. The conduction band and valence band are still discontinuous, intensifying the band bending.
In the pn junction, the uneven distribution of carrier concentrations in the two materials leads to a directional shift of the carriers from the place of high concentration to the area of low concentration. The electrons in Bi2O2Se move to the WSe2 side, leaving immovable positive-charged ionized donors who lose their equilibrium negative charge and thus form a positive charge area on the N side near the pn junction. Similarly, a negatively charged area consisting of ionized acceptors appears on the side of the p-region near the pn junction. These charges in the space charge region generate a built-in electric field pointing from the Bi2O2Se side towards WSe2. In the absence of external source−drain voltage and gate voltage, photogenerated electron−hole pairs are generated on the surface of Bi2O2Se by 640 nm monochromatic excitation. Recombination occurs when they do not separate into the junction region. Due to the presence of in-plane heterojunctions between WSe2 near the junction and WSe2 away from the junction, photocurrents appear around the junction region rather than in the upper layer. Under the internal electric field, the electrons move to the side of Bi2O2Se, and the holes move to the side of WSe2, forming a photocurrent from Bi2O2Se to WSe2.
If a positive gate voltage (Bi2O2Se to WSe2) is applied, the gate injects electrons through the gate medium into the heterojunction. The potential energy of the electrons in the conduction band and valence band decreases overall, resulting in a downward shift of the conduction band and valence band relative to the Fermi level. If the Vg is high enough to provide a large enough electric field, the direction of the electric field can be changed, which is described in Fig. 5(a) for this extreme condition. If a positive source−drain voltage (Vds) is added to the positive Vg, the potential energy on the Bi2O2Se side will increase, causing the energy band to shift upward, as shown in Fig. 5(b). If enough positive bias is added, the energy of the electrons in the junction will be higher than the barrier energy. In other words, electrons are more likely to cross the barrier to reach the WSe2 side, and the higher the gate voltage, the more likely they are to jump. Under the superposition of Vg and Vds, the direction of the total electric field is from WSe2 to Bi2O2Se. At this time, photogenerated carriers are generated in the material by the excitation beam. Under the action of the applied electric field, the holes excited by the light migrate from WSe2 to the source, and the electrons in Bi2O2Se diffuse to the drain. As a result, the photocurrents from WSe2 to Bi2O2Se are in the Fig. 6(c). Fig. 6(a) shows the photocurrent scan area, and Fig. 6(b) shows zero gate voltage and source−drain voltage. As shown in Fig. 5(c), when Vds < 0, the opposite direction of the electric field causes the light-generated carriers to migrate in the opposite direction. The carrier concentration is low, the photocurrent is weak, and the photocurrent pattern corresponds to Fig. 6(d).
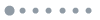
Figure 5.(Color online) Band diagrams of the junction with photoexcitation under the different Vds and Vg, which reflected the charge transport process.
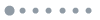
Figure 6.(Color online) Photocurrent map of the Bi2O2Se/WSe2 heterojunction field-effect transistor. (a) Bi2O2Se/WSe2 heterojunction optical images, the white box indicates scanning area; (b) Vds = 0 V, Vg = 0 V; (c) Vds = 3 V, Vg = 40 V; (d) Vg = −3 V, Vds = 40 V; (e) Vds = 3 V, Vg = −40 V; (f) Vds = −3 V, Vg = −40 V.
In the case of a negative gate voltage, the band shifts upwards concerning the Fermi level as a whole, as shown in Fig. 5(d). In Fig. 5(e), the electron energy on the Bi2O2Se side decreases at Vds > 0 compared to the Vg > 0 time, and the potential barrier increases, making it difficult for the electrons to transition. In this case, the I−V curve appears to be turned off and a pulsed photocurrent appears, as shown in Fig. 6(e). When the source−leakage voltage Vds is negative, a large total electric field is obtained by superimposing Vg and Vds in the same direction as the built-in electric field, resulting in severe band bending and a very narrow barrier, which may lead to direct tunneling, as shown in Fig. 5(f). As a result, the Bi2O2Se/WSe2 heterojunction FETs are in a conductive state and the photocurrent is significantly increased, as shown in Fig. 6(f). Under illumination, the difference in work function between Bi2O2Se and WSe2 leads to the formation of an built-in electric field between Bi2O2Se and WSe2, and the presence of the built-in electric field is conducive to the separation of electrons and holes so that the heterojunction region has a powerful light intensity. Compared with horizontal heterojunctions, the photocurrents generated by vertical heterojunctions are not obvious, which is due to the absorption of light by the thicker Bi2O2Se in the junction region. However, in the case of the tunneling effect, the photocurrent is so strong that it covers the entire junction region.
When 640 nm monochromatic light irradiates the surface of the heterojunction, Bi2O2Se and WSe2 are excited to form electron−hole pairs, which effectively separate under the action of the built-in electric field. And move in different directions to generate photocurrent. As the light intensity increases, the photocurrent in the transistor increases, as shown in Fig. 7(a). Due to the narrow bandgap of Bi2O2Se and the wide bandgap of WSe2, the optical response range of the Bi2O2Se/WSe2 heterojunction FET in Fig. 7(b) can cover the visible near-infrared region. Under monochromatic excitation at a wavelength of 380 nm, the maximum photocurrent value can reach 176 μA. Even in the near-infrared region, where the response is minimal, the photocurrent value can attain 11 μA. Meanwhile, it also confirms the analysis above, that the photocurrent is mainly contributed by the light absorption on the side of WSe2, and then the photogenerated carriers are separated in the junction region. If Bi2O2Se is the main contribution, the response range can be extended to about 1550 nm[14]. The switching characteristics of the photocurrent of the device are in Fig. 7(c). At a wavelength of 640 nm and a power density of 1.27 mW/cm2. The switching characteristics of the transistor are evident, with rise and fall times of 58 and 40 μs, respectively. The rise time is generally defined as the time to rise from 10% to 90% of the waveform, and the fall time is reversed. The recombination rate of the carriers is faster than the rate of generation, which we attribute to the inherent defects of the material. Bi2O2Se/WSe2 heterojunction generates photogenerated carriers in the junction region, and then the electrons and holes are separated until they are combined in the outer circuit, thus completing a photoelectric response. Due to the high mobility of Bi2O2Se, multiple carrier cycles on the Bi2O2Se side produce a gain, the device has a high responsivity of about 1500 mA/W. The Bi2O2Se/WSe2 heterojunction transistors have an advantage in terms of comprehensive performance in response time and responsivity, both when compared to WSe2 transistors prepared in different ways[23−25], and when compared to other WSe2-based heterojunction transistors previously reported[11, 26−28]. In addition, the detectivity of our device can reach 1.65 × 107 jones. Our results demonstrate the potential of Bi2O2Se/WSe2 heterojunction FET in the fields of bidirectional rectification and fast response.
![(Color online) (a) Output curves of Bi2O2Se/WSe2 heterojunctions under different light power irradiation. (b) Spectral response of the heterojunctions. (c) Response time of the device over one response cycle. (d) Composite comparison diagram of photoresponse performance among WSe2-based photodetectors[11, 23−28].](/Images/icon/loading.gif)
Figure 7.(Color online) (a) Output curves of Bi2O2Se/WSe2 heterojunctions under different light power irradiation. (b) Spectral response of the heterojunctions. (c) Response time of the device over one response cycle. (d) Composite comparison diagram of photoresponse performance among WSe2-based photodetectors[11, 23−28].
4. Conclusion
In summary, we accurately aligned the multilayer WSe2 crystals prepared by mechanical exfoliation with Bi2O2Se grown by chemical vapor deposition to construct the Bi2O2Se/WSe2 heterojunction FET, which not only exhibits basic diode characteristics but can also be back-biased under the control of gate voltage, the rectification ratio of the device can approach 105. Under different gate voltage modulation, the current on/off ratio of the device changes by nearly five orders of magnitude, meanwhile the maximum current on/off ratio of the device has outdistanced 105. The work functions and band alignment obtained by KPFM and UPS are consistent with type Ⅱ heterojunction characteristics. The conjecture that the direction of the electric field in the heterojunction depends on the inversion of the gate voltage is verified by the interpretation of the photocurrent diagram. The on/off of the device is controlled by adjusting the gate voltage and the source−drain voltage, and the related mechanisms are described in detail. In addition, the device has excellent optoelectronic characteristics, with a spectral response from visible light to the near-infrared region, and the rise and fall times of the light response in the order of microseconds within a single light response cycle. Therefore, the device has a gate voltage regulation function and unique optoelectronic characteristics, which is expected to broaden the application of two-dimensional material heterojunction devices in the field of optoelectronics, and take a significant step for the development of high-performance bidirectional rectifiers concurrently.