Introduction
Transition metal dichalcogenides (TMD) such as, molybdenum disulfide, tungsten disulfide (WS2), molybdenum diselenide, and tungsten diselenide, are two-dimensional (2D) layered semiconducting materials with the bandgap energy of visible and infrared light. In monolayer (1L) thickness, these TMD are direct bandgap semiconductors, exhibiting robust light-matter interactions, with the distinct photoluminescence (PL)1. 1L-TMDs display low PL quantum yield (PLQY) due to the high density of lattice defects2−6 and PLQY of 1L-TMD are especially low under ultra-violet (UV) illumination because UV light mostly excites the carriers at so-called band nesting region located between Γ and points of the 1st Brillouin zone, which mostly decay non-radiatively before reaching to the point where the bandgap transition occurs7,8. Extensive studies have been carried out to improve the PLQY of 1L-TMD with some promising successes9,10, however the enhancement of PLQY upon UV excitation has rarely been studied, severely hampering the practical use of 1L-TMD in UV optoelectronic devices11,12.
In recent decades, extensive research has been devoted to zero-dimensional (0D) quantum dots (QD) derived from atomically thin 2D materials, including graphene, MXene, graphitic carbon nitride (GCN), hexagonal boron nitride, and phosphorene13. The intriguing optical, electronic, and chemical features of these 2D/QD have enabled a vast array of applications in optoelectronics, photocatalysis, supercapacitors, photovoltaics, biosensors, and energy storage13−17. When 2D materials are fragmented down to a few nanometers of lateral sizes, improved and distinct characteristics emerge because of prominent edge and quantum confinement effects, while retaining inherent merits of their 2D precursor13,14,18. Furthermore, these 2D material-derived QD feature a larger surface-to-volume ratio, better solubility in both aqueous and nonaqueous solvents, higher tunability in physicochemical properties, increased flexibility to hybridize with other nanomaterials, and the easier doping and functionalization than their native 2D forms19. Especially, MXene-derived QD (MQD) and GCN-derived QD (GCNQD) with their intrinsic advantages of environmentally friendly chemical components have been documented to possess exceptional optical properties, notably a broad range of energy absorption down to the deep UV region and efficient blue fluorescence20,21. In recent years, the construction of 0D/2D mixed-dimensional hybrid has effectively enhanced the PL intensity of TMD as a consequence of interfacial energy transfer (ET) in the hybrid structures22,23. For instance, Yu et al. reported a 13-fold enhancement in the PL intensity of 1L-WS2 dispersed with CsPbBr3 QD under 405 laser excitation22−24. Su et al. showed an enhanced non-radiative resonant ET efficiency up to 73% for hybridization of 1L-WS2 with carbon QD by varying the level of nitridization under visible laser excitations25. Also, Su et al. demonstrated full-color, tunable emission in 1L-MoS2/QD thin films prepared from graphene oxide QD or graphene QD26. However, these investigations focused on the optical behavior of 1L-TMD under the illumination using visible-light wavelength ranging from 405 to 532 . To date, the PL enhancement in 2D TMD by using QD hybridization under UV illumination has not been reported.
In this work, titanium nitride (Ti2N) MQD and GCNQD having efficient UV absorption were independently incorporated into 1L-WS2 to develop 0D/2D hybrid structures. Herein, we take full advantage of the merits of these QD facilitating 1L-WS2 to harvest UV light and boost their inherently low PLQY under UV illumination7,8, which to our knowledge have not been explored yet. Additionally, the MQD and GCNQD are environmentally friendly, distinguished from conventional inorganic QD making them suitable for various biomedical applications21,27. We conclude that the intra-hybrid ET from the donor MQD or GCNQD to the acceptor 1L-WS2 is chiefly responsible for the PL enhancement observed in the 1L-WS2/QD hybrid. Accordingly, we combined the efficient UV response of the QD with the excellent optical properties of 1L-WS2 in the visible range, suggesting a fascinating approach for strengthening the optical performance of 1L-TMD in the UV energy range.
Experimental
Preparation of 1L-WS2
High-quality WS2 (HQ graphene) was mechanically exfoliated from bulk WS2. Initially, the 1L-WS2 crystals were stripped from the bulk laminate using a transparent Scotch tape and peeled repeatedly with blue Scotch tape. Afterwards, they were transferred from the blue tape to a polydimethylsiloxane (PDMS) film which was attached to the glass slide, and gently detached after a short rest period to ensure that the 1L-WS2 crystals adhered abundantly to the PDMS film2,28,29. Eventually, the 1L-WS2 flakes deposited on top of the PDMS stamp were examined under an optical microscope to locate the 1L-WS2 according to the optical contrast under bright-field illumination.
Synthesis of MQD and GCNQD
The MQD were prepared from Ti2N MXene powder using a combination of sonication and hydrothermal methods, as reported by Anir et al30. Briefly, Ti2N MXene powder (1.1 ) was obtained by etching the Al layer from the titanium aluminum nitride (Ti2AlN) MAX phase, which was then mixed with deionized water (10 ). After that, a few drops of ammonium hydroxide (NH4OH) were added slowly to the mixture and subjected to heating at 100 °C for 6 hours. The resulting mixture was then centrifuged at 10000 and the supernatant of the Ti2N MQD was collected. In addition, the GCNQD were synthesized based on the ethanol-thermal treatment approach described by Zhan et al21. Concisely, urea precursor (20 ) was heated at 550 °C for 6 h with a ramp rate of 3 °C min−1. Subsequently, as-synthesized bulk GCN powder (0.03 ) was dispersed in ethanol (30 ), and concentrated potassium hydroxide (0.45 ) was added. Afterwards, the mixture was kept in an oven for 6 at 180 °C, followed by centrifugation at 12000 . Finally, the as-obtained GCNQD pellet was redispersed in water and filtered using a 0.2 syringe filter. The transmission electron microscopy (TEM) image in Fig. S1 shows the size distribution of Ti2N MQD and GCNQD to be 3.2 and 3.5 respectively. We further analyzed the element composition of both QD with X-ray photoelectron spectroscopy (XPS) in Fig. S2.
Preparation of 1L-WS2/QD hybrid
A few drops of diluted QD, either MQD or GCNQD were dispersed on the cleaned SiO2/Si substrate and dried in the vacuum oven at 100 °C. Subsequently, the thin film of QD formed on the SiO2/Si substrate was scratched using a stainless-steel ruler to create an array of QD-dispersed and clean region without QD. Following that, exfoliated 1L-WS2 were deposited across the QD-dispersed and clean region without QD substrate surfaces using a dry transfer approach. The samples were then thermally treated in a vacuum oven to strengthen the adhesion between the 1L-WS2 and the QD.
Materials characterizations
The morphologies of the Ti2N MQD and GCNQD were analyzed using high-resolution TEM (JEM-3010, JEOL) and atomic force microscopy (AFM) (XE-120, Park Systems). Ultraviolet photoelectron spectroscopy (UPS) and XPS were conducted using an ESCALAB 250Xi with a monochromatic Al X-ray line. The UV-Vis absorption and PL spectra of the QD solutions were examined using an absorption spectrometer (Optizen, K Lab) and a fluorescence spectrophotometer (Cary Eclipse, Agilent), respectively. The confocal PL mappings were performed using a lab-built laser confocal microscope upon photoexcitation of 375 , 405 and 514 continuous-wave laser at low excitation powers of approximately 76 μW, 17 μW, and 0.8 μW, respectively, measured at the sample. The laser light was focused using a 100× objective lens (NA = 0.95) and guided to a 50 long monochromator equipped with a cooled charge-coupled device (CCD) (PIXIS 400, Princeton Instruments). For the optical absorption measurements, a commercial confocal microscope (Alpha-300S, WITec Instrument GmbH) with an inverted 60× objective lens was employed. Epifluorescence images of the hybrid samples were captured using a cooled electron-multiplying CCD camera (Photon MAX 512, Princeton Instruments) in a low-light environment with an exposure time of 60 s. A 300 UV LED was used as the excitation light source. The UV light was focused using 20×, 0.39 NA micro spot UV focusing objectives and the scattered light was collected using the same objective lens (optical layout of the UV epifluorescence is shown in Fig. S3 in the Supplementary information). The measurement of PL decay lifetime was conducted using the same confocal microscope under the excitation of a 375 picosecond pulsed diode laser (BDL-375, Becker & Hickl GmbH). The PL lifetime data were collected using a time-correlated single-photon counting (TCSPC, Becker & Hickl GmbH) correlator. The PL emission of the QD were obtained by selectively filtering out the PL emission from 1L-WS2 in the wavelength range of 560–680 using a 550 short-pass filter. All optical measurements were conducted at 300 K.
Results and discussion
PL characterization of 1L-WS2/MQD hybrid under UV and visible light illuminations
The schematic in Fig. 1(a) illustrates the hybrid of 1L-WS2 and MQD, or GCNQD dispersed on the SiO2/Si substrate. The optical microscope image in Fig. 1(b) shows a 1L-WS2 flake placed across the boundary between the MQD-dispersed and the clean region without MQD on the SiO2/Si substrate. We checked the Raman spectra of our 1L-WS2 (Fig. S4) and the peak difference of 63.9 between A1g and E2g modes was consistent with the 1L thickness of 1L-WS231. Here 1L-WS2 was exfoliated from bulk WS2 as one piece, and thus, some parts of them were seemingly cracked during the transfer process, but PL characteristics such as the intensity or peak positions of the 1L-WS2 flake shown in the image are expected to be spatially homogeneous. Therefore, any variation in the PL emission of 1L-WS2 across the boundary between the QD region and clean region without QD can be regarded as a result of QD hybridization. Fig. 1(c) and 1(d) display the confocal PL intensity mapping of the same area shown in Fig. 1(b) with two different laser excitation wavelengths () of and , respectively. Clearly, with , PL intensity of 1L-WS2 is distinctively stronger at 1L-WS2/MQD than at 1L-WS2 without MQD. PL spectra averaged from the entire region of the 1L-WS2/MQD showed a 4.3 times enhancement compared to the 1L-WS2 without MQD hybridization, as shown in Fig. 1(e). The same area of the sample with showed that the PL of 1L-WS2/MQD was somewhat weaker than that of 1L-WS2. The average PL spectra with also confirmed the slight reduction (0.7 times) of the PL intensity of 1L-WS2 upon hybridization with MQD as shown in Fig. 1(f). We inspected several samples in the similar sample configuration and found that the PL of 1L-WS2/MQD was much enhanced with but slightly reduced with (Fig. S5).
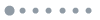
Figure 1.(a) Schematic diagram illustrating the hybrid structure of 2D/QD and 1L-WS2. (b) Optical image and (c, d) Confocal PL mapping images of the 1L-WS2 and 1L-WS2/MQD hybrid obtained with 375 and 514 , respectively. Scale bar is 8 . (e, f) Representative PL spectra of 1L-WS2/MQD (red curve) and 1L-WS2 (blue curve) with 375 and 514 .
Optical characterization of 1L-WS2/MQD hybrid under 300 nm UV LED illumination
Based on the aforementioned results of the PL enhancement of 1L-WS2 by in Fig. 1, we expected that the PL of 1L-WS2 by MQD hybridization could exhibit even higher PL enhancement if used with a shorter of UV illumination. Figure 2(a) and 2(b) show the optical view of 1L-WS2 flake partly sitting on the dispersed MQD and its epifluorescence image obtained with of UV excitation, respectively (the experimental layout of the deep-UV epifluorescence imaging is given in the Supplementary information (Fig. S3)). The PL intensity of 1L-WS2 on MQD was noticeably higher than that on the area without MQD. Figure 2(c) shows that the PL intensity of 1L-WS2/MQD is nearly 15 times higher than that of 1L-WS2 without MQD.
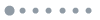
Figure 2.(a) Optical microscope image and (b) Epi-fluorescence image of 1L-WS2/MQD hybrid under λex=300 nm UV illumination (orange dotted lines in A indicates the boundary of MQD region and clean region without MQD). Scale bar is 2 μm. (c) Representative PL spectra of 1L-WS2/MQD hybrid (red curve) and 1L-WS2 (blue curve) showing a 15-fold enhancement in PL.
Optical characterization of 1L-WS2/GCNQD hybrid
In Fig. 3, we show the results of PL mapping of 1L-WS2 hybridized with GCNQD. The confocal PL intensity maps of 1L-WS2 partially sitting on the GCNQD are shown in Fig. 3(a) and 3(b), with two different wavelengths of laser excitation at 375 and 514 , respectively. Similar to the results for 1L-WS2/MQD, the PL of 1L-WS2/GCNQD was greatly enhanced compared to 1L-WS2 with 375 while the enhancement was not distinct with . A comparison of the average PL spectra also confirmed the same trend of PL enhancement by 3.4 times with hybridization with GCNQD under (Fig. 3(c)), whereas the enhancement was not significant with (Fig. 3(d)). We also used 300 wavelength light excitation, as the epi-fluorescence image and PL spectra are shown in Fig. 3(e) and 3(f), respectively, where 1L-WS2/GCNQD exhibited almost 11 times enhancement of PL. We believe that the light absorption by 1L-WS2 in 1L-WS2/QD hybrid is expected to be similar to that of 1L-WS2, thus the observed PL enhancement of 1L-WS2/QD hybrid could be directly interpreted as the enhancement of the PL efficiency or PLQY of 1L-WS2.
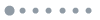
Figure 3.(a, b) Confocal PL mapping images of 1L-WS2/GCNQD hybrid with of 375 and 514 , respectively. Scale bar is 8 μm. (c, d) Representative PL spectra of 1L-WS2/GCNQD (red curve) and 1L-WS2 (blue curve) with λex=375 nm and λex=514 nm, respectively. (e) Epi-fluorescence image of 1L-WS2/GCNQD hybrid with λex=300 nm. (f) Representative PL spectra of 1L-WS2/GCNQD (red curve) and 1L-WS2 (blue curve) with λex=300 nm.
Absorption enhancement of 1L-WS2/MQD and 1L-WS2/GCNQD at UV range
We studied the absorption characteristics to investigate the origin of the PL enhancement observed in 1L-WS2/QD. While the continuous micro absorption spectra of 1L-WS2 or 1L-WS2/QD can be obtained for the visible wavelength range32, we were able to obtain the absorption at two fixed UV wavelengths by using the fixed wavelength of laser excitation at 300 and . In Fig. 4, the absorption spectra and data points of 1L-WS2, 1L-WS2/MQD and 1L-WS2/GCNQD are provided that are obtained using the micro-absorption mapping technique32. We note that the absorption of 1L-WS2/QD is mostly the same as the absorption of 1L-WS2 for the wavelength longer than 450 , then it steeply enhanced compared to the absorption of 1L-WS2 as the wavelength goes into the UV region. The absorption of 1L-WS2/MQD (1L-WS2/GCNQD) obtained at 300 and 375 showed 8.0 (7.6) and 2.6 (5.3) times-increase compared to 1L-WS2, respectively. This increased absorption at UV range is attributed to efficient absorption of UV light by MQD and GCNQD having wide bandgap energies of 3.8 30 and 3.4 33 respectively (Absorption and PL spectra of the MQD and GCNQD are shown in Fig. S6 in the Supplementary information). We believe that the efficient absorption of UV light by QDs in 1L-WS2/QDs hybrids must have contributed to the increase of the PL of 1L-WS2 in 1L-WS2/QDs hybrids through the ET process, as the detailed mechanism of ET is discussed in following section.
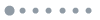
Figure 4.Measured micro absorption spectra of 1L-WS2/GCNQD (black curve), 1L-WS2/MQD (blue curve) and 1L-WS2 only (red curve) as a function of photon wavelength. Six discrete data points are measured absorptions of 1L-WS2/GCNQD (black), 1L-WS2/MQD (blue) and 1L-WS2 only (red) measured by using laser sources at 300 nm and 375 nm wavelengths. Dashed lines are guides for the eyes.
TRPL and energy band alignment of 1L-WS2/QD hybrid
On contrary to the greatly enhanced PL from 1L-WS2 in 1L-WS2/QD hybrid under UV excitation, the PL of MQD and GCNQD in the 1L-WS2/QD hybrid were observed to be significantly quenched by four and three times, respectively, compared to the bare QD as the representative PL spectra are shown in Fig. 5(a) and 5(b). We also obtained time-resolved photoluminescence (TRPL) curves and commonly observed the reduction of PL lifetime of QD, from 1.06 to 0.6 and from 1.33 to 1.0 for MQD and GCNQD with hybridization with 1L-WS2, respectively, as shown in Fig. 5(c) and 5(d). Table S1 in the Supplementary information shows the value of short and long components of the decay fitting function. Such PL quenching and reduction of the PL lifetime of QD, together with the PL enhancement of 1L-WS2 for 1L-WS2/QD hybrid upon UV irradiation as discussed above, strongly suggest the occurrence of ET from QD to 1L-WS2 in both of 1L-WS2/MQD or 1L-WS2/GCNQD hybrid upon UV excitation. ET, a non-radiative transfer of energy from one quantum system in excited state (doner) to the other in the ground state (acceptor) and ET between 1L-TMDs and QDs were previously reported34 We also observed a slight reduction of PL lifetime of 1L-WS2 emission with hybridization of QD, as the result are shown in Fig. S7 in the Supplementary information. Such reduction of emission lifetime of the acceptor during ET were previously reported35,36. Figure 5(e) schematically describes the energy levels of conduction band minimum () and the valence band maximum () and the Fermi level () of 1L-WS237,38, Ti2N MQD30, and GCNQD33 as estimated from the (UPS) measurement (Fig. S8 in the Supplementary information), bandgap energy estimated from the absorption measurement and the values reported in the literature39. Both 1L-WS2/MQD and 1L-WS2/GCNQD are expected to form Type I band alignment between the MQD (or GCNQD) and 1L-WS240, and we note that PL spectrum of the MQD (and GCNQD) show considerable spectral overlap with the absorption spectrum of 1L-WS2 as shown in Fig. S9, which is a favorable condition for ET to occur in our 1L-WS2/QD hybrid. Thus, we strongly believe that the significant portion of absorbed energy in MQD and GCNQD in 1L-WS2/QD hybrid under UV irradiation were transferred to 1L-WS2 causing the PL enhancement of 1L-WS2.
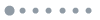
Figure 5.PL spectra obtained from (a) MQD and (b) GCNQD in hybrid of 1L-WS2/QD. (c) Time-resolved photoluminescence (TRPL) of MQD emission of isolated MQD (blue curve) and 1L-WS2/MQD hybrid (orange curve). (d) TRPL of GCNQD emission of isolated GCNQD (green curve) and 1L-WS2/GCNQD hybrid (purple curve) at λex=375 nm. Dotted line represents the fitting curve. The emission of (c) and (d) was collected at the wavelength range of 400-550 nm using the combination of a long-pass filter and a short-pass filter to exclude the emission of 1L-WS2. (e) Schematic of energy band alignment showing the type I band alignment of hybrid structure between QD and 1L-WS2. Values of the conduction band minimum (ECBM) and the valence band maximum (EVBM) and the Fermi level (EF) for each material are marked.
In addition to ET, charge transfer (CT) at the interface between 1L-TMD and QD should be also considered because 1L-WS2 and MQD or GCNQD are making a quantum interface and electrons or holes can efficiently transfer across the interface as previously reported in similar 1L-TMD/QD hybrids41,42, and CT has a major effect on the PL emission of 1L-TMD24,43, because the PL efficiency of 1L-TMD is heavily affected by excess charge density44,45. In 1L-WS2 that is intrinsically n-type46,47, electron transfer to 1L-WS2 causes an increase in the trion (A-) spectral weight over the neutral exciton (A0). Because A- tends to decay non-radiatively and has less energy of emission than neutral excitons, increased portion of A- usually causes an intensity reduction and the red-shift of A peak, respectively41,48. We deconvoluted the representative PL spectra of 1L-WS2/MQD and found that under , the A- portion increased from 27% to 45%, suggesting the transfer of electrons from MQD to 1L-WS2 when excited by near-bandgap excitation of MQD (). 1L-WS2/GCNQD showed a similar pattern in which the A- portion was increased from 32% to 45% under. (Deconvolution of the PL spectra of 1L-WS2/MQD into A0 and A- is shown in Fig. S10 in the Supplementary information). The increase of electron density in 1L-WS2 observed from both 1L-WS2/MQD and 1L-WS2/GCNQD is attributed to the CT of photo-excited electrons from QD to 1L-WS2, because the energy of 375 wavelength laser light (3.3 ) is high enough to excite QD and the conduction band of 1L-WS2 is lower than that of Ti2N MQD or GCNQD. Despite the increased A- portion of the PL of 1L-WS2 in 1L-WS2/QD hybrid, which generally gives rise to PL quenching49, we observed a significant increase in the emission intensity under UV illumination. This indicates that the ET from QD to 1L-WS2 is the dominant effect to enhance the PL of 1L-WS2. Considering the efficiency of CT may be controlled by the distance between the doner and acceptor materials, we expect the use of atomic thickness dielectric spacer such as hBN layer could mitigate the CT effect further improving the PL enhancement50,51.
In order to further investigate the CT in 1L-WS2/QD hybrid, we performed the Raman measurement as the result are shown in Fig. S11 in the Supplementary information. We found that with where the photon energy is less than the bandgap of MQD or GCNQD A1g Raman peak in the 1L-WS2/MQD was red-shifted by ~2 , suggesting the increase of electron density in 1L-WS252 by hybridization with MQD. For 1L-WS2/GCNQD, slight blue-shift of the A1g peak by ~1 was detected suggesting the electron depletion of 1L-WS2 by GCNQD. Such n-doping and p-doping effects of 1L-WS2 by MQD and GCNQD suggested from Raman spectra, respectively, are consistent with the increase and decrease of A- spectral weights of PL spectra taken with as shown in Fig. S10. We note that at , charge carriers of QD are not excited either in 1L-WS2/MQD or 1L-WS2/GCNQD, limiting the electron transfer from QD to 1L-WS2. Thus, observed variations of PL spectral weights and Raman A1g peak positions are attributed to the CT due to the slight differences in Fermi level between 1L-WS2 and MQD or GCNQD, as shown in Fig. 5(e).
Conclusion
We showed that hybridization with Ti2N MQD and GCNQD gave rise to a maximum of 15 times increase in the PL emission of 1L-WS2 for 300 wavelength of UV excitation. The observed PL enhancement in the 1L-WS2/QD hybrid strongly suggests the occurrence of ET from the MQD or GCNQD to 1L-WS2 upon UV excitation, as evidenced by TRPL, Raman and PL analysis. The increase of A- spectral weight and red-shift of A peak position suggest that the CT also occurred in the 1L-WS2/QD hybrid which can cause the PL quenching under visible light. However, the PL enhancement was still observed because the contribution of increase in absorption and the energy transfer under UV illumination compensated the undesirable CT effect. Because ET and CT are crucially dependent on the alignment of energy bands, use of various kinds of 1L-TMDs to hybridize with MQD or GCNQD can provide diverse characteristics in terms of the enhancement and tunability of light emission from 1L-TMDs, which are to be further investigated in separate studies. Our findings offer an efficient approach for enhancing the UV response of 1L-WS2 with the hybridization using wide-bandgap QD, thus paving the way for the development of next-generation optoelectronic and fluorescence-sensing probe technologies with superior UV light-harvesting performances using 1L-TMD.