Introduction
The growing popularity of AI, internet of things, cloud computing, and multimedia has increased the demand for optical communication with high transmission rates, large bandwidths, long distances, high security, low loss, EMI resistance, and low cost[1, 2]. Integrated photonics mainly relies on two platforms: indium phosphide (InP) and silicon photonics (SiPh). InP platforms can integrate both passive and active components, like lasers, amplifiers, and photodetectors[3]. However, SiPh has a distinct advantage over InP in its compatibility with advanced CMOS technologies, leading to lower costs and higher production volumes. SiPh is used in terahertz generation, high rate signal detecting, potential neuromorphic computing[4], optical switches[5, 6], light detection and ranging (LIDAR)[7−9], and optical sensing[10−12]. Photodetectors, converting optical signals into electrical ones, are crucial for the wide spread application of 1310 nm optical communication.
Currently, ethernet systems with capacities such as 400 G/800 GBASE and beyond are essential for supporting rapidly expanding applications. For example, while current 4 × 100 Gb/s photodiode (PD) receivers suit 10 km applications, their range drops to 2 km when data rates reach to 200 Gb/s per lane[12−14]. Moreover, as relay distances and the number of access network users increase, link losses reduce the optical power at the user end, necessitating higher sensitivity. Consequently, p−i−n and metal−semiconductor−metal (MSM) photodetectors cannot meet high-rate requirements[15−18]. APDs featuring an avalanche region significantly enhance receiver sensitivity[19]. The impact ionization ratio, affecting sensitivity and reducing excess noise, ranges from 0.01 to 0.1 in silicon. This makes Ge-on-Si APDs perform better than most Ⅲ−Ⅴ APDs[20−23]. Furthermore, Ge-on-Si APDs are compatible with CMOS technology and cost-effective, thanks to their raw material composition.
Recent efforts have aimed at enhancing Ge-on-Si APDs' performance. For instance, Huang et al. added an silicon layer to control the electrical field distribution at O-band, the result shows that 3dB-bandwidth is 56 GHz, and the responsivity is 1.08 A/W[24]. Zeng et al. studied the impact of voltages and input powers on 3-dB bandwidth, employing an effective equivalent voltage for a quantitative analysis[25]. Zhang et al. discovered that surface defects and strong electric fields at the sidewalls lead to significant leakage currents[26]. SACM Ge-on-Si APDs get more attention. Studies show that vertical SACM Ge-on-Si APDs operate effectively at voltages below −10 V[22, 27, 28]. Fabricating these APDs involves silicon epitaxial growth and the addition of metal electrodes in the germanium layer which will reduce the primary responsivity. In contrast, lateral SACM Ge-on-Si APDs are emerging, eliminating the need for silicon epitaxy growth and metal contact steps. Some studies have focused on increasing the device's bandwidth by etching shallow trenches on the left and right sides of the Ge[29−31]. These devices exhibit low responsivity at unit gain and high dark current. Additionally, controlling the doping region for designs narrower than 100 nm is challenging[30]. Efforts to improve absorption efficiency have achieved a 3-dB bandwidth of 48 GHz and a high primary responsivity of 0.93 A/W[32]. Furthermore, Li et al. have extended the 3-dB bandwidth to 75 GHz using inductive-gain-peaking technology[33]. Lischke et al. utilized a Fin structure in germanium to achieve a 3-dB bandwidth to 265 GHz[4]. Chowdhury et al. demonstrated a lateral SACM Ge-on-Si APD whose responsivity at the bias voltage of 5 V is 26 A/W. Peng et al. showcased all-Si 20 Gb/s avalanche photodiodes with a responsivity exceeding 65 A/W[34]. Zhang et al. were the first to use an InGaAs/InAlAs APD on a silicon platform, achieving a gain exceeding 70 at 1570 nm[35].
This paper introduces a lateral SACM Ge-on-Si APD. Designed for compatibility with the CMOS process at 1310 nm, the device utilizes a simplified standard process. Experiments were conducted to measure both the photocurrent and dark current of the detector. Responsivity and gain were meticulously calculated. Additionally, comprehensive analyses of bandwidth and eye diagrams were conducted. The results show a unit-gain responsivity of 0.5 A/W for the APD. At a 15 V bias voltage, responsivities of 2.98, 3.11, 3.01, and 2.91 A/W were observed for input optical powers of −10, −15, −20, and −25 dBm, respectively. Additionally, the 3-dB bandwidth at high bias voltages was discussed. Finally, the eye diagrams at 20 and 10 Gbps are obtained.
Design and fabrication
Fig. 1 illustrates a 3D schematic and top layer layout of the waveguide-integrated lateral SACM Ge-on-Si APD structure on a 0.18 μm silicon platform, featuring a p-type <100> top Si layer with a 220 nm layer and a 3 μm thick buried oxide (BOX). The SACM structure is designed because Ge's bandgap matches the 1310 nm optical communication band, and silicon's ionization coefficient is much less than 1, reducing device noise and increasing the gain-bandwidth product. Ideally, the electric field should be maintained between 1 × 104 and 2 × 105 V/cm. If too high, it causes the Ge layer to multiply and produce excess noise; if too low, it affects the drift of photogenerated carriers in silicon at the saturation rate[36, 37]. The 0.2 μm wide multiplication region is located between the Pul (P-type ultra-low doping) charge layer and the P-type doped region. A Ge-epi layer, 0.5 μm wide and 10 μm long, serves as the absorption layer. For enhanced visual clarity, a portion of the device's structure is magnified in Fig. 1(b).
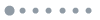
Figure 1.(Color online) (a) 3D perspective view for SACM Ge-on-Si APD; (b) top-down perspective of the mask layout in the fabrication process for the device.
Fig. 2 illustrates the vital fabrication steps of the device using the 180 nm MPW process. The APD design process can be summarized as follows: Firstly, in Fig. 2(a), the channel waveguide and grating were created through dry etching to depths of 220 and 70 nm, respectively. To support single mode, the strip waveguide's width is designed to be 450 nm. To reduce scattering loss and increase waveguide edge smoothness, high temperature annealing is required after etching[38, 39]. The period and duty of the designed grating are 490 nm and 0.5, respectively. Secondly, Fig. 2(b) shows that five-step ion implantation completes the charge layer and ohmic contact doping. The doping dose of the charge region is 1.2 × 1012 cm−2 with a width of it is 0.25 μm, designed to enlarge the high electric field in silicon layer. P-ohmic and n-ohmic doping, with boron and phosphorus respectively, involve three steps each, with doping dose of 1012−1015 cm−2. Thirdly, preparation of germanium epitaxy in Figs. 2(c) and 2(d). After growing a polyethylene oxide (PEOX) layer, rapid thermal annealing (RTA) activates and repairs injected impurities and any injection-induced damage. A 500 nm diameter circular hole is created through lithography and etching. Fourthly, after cleaning, the reduced pressure chemical vapor deposition (RPCVD) growth method is typically used to deposit Ge[40, 41]. The depths of the Ge layer and the waveguide are 365.2 and 220 nm, respectively. Due to the uneven thin film layer, chemical mechanical polishing (CMP) is necessary to smooth the Ge epitaxial surface. Due to the device's lateral structure, there is no need for ion doping on germanium. Additionally, the lateral structure's lack of contact electrodes simplifies the process and prevents light signal scattering and absorption by electrodes in germanium. Fifthly, tungsten plug and AlCu preparation can be seen in Fig. 2(e). After cleaning, a Ni film is deposited inside the contact hole by physical sputtering. High-temperature annealing then forms a nickel-germanium alloy to reduce contact resistance. A Ti/TiN layer is also deposited to enhance adhesion between the tungsten plug and the hole's inner wall, preventing tungsten diffusion. Since tungsten is difficult to remove by plasma, CMP technology is used for its removal, forming the tungsten plug. Afterward, the wafer is transferred to the PVD equipment for AlCu alloy deposition, followed by a TiN alloy layer to prevent aluminum electromigration and serve as a reflective layer for lithography. Sixthly, M2 completion is shown in Fig. 2(f). Finally, the wafer is annealed at 400 °C in an anaerobic nitrogen atmosphere.
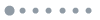
Figure 2.(Color online) The main steps for waveguide-coupled lateral SACM Ge-on-Si APD. (a) Etching waveguides and gratings on the top 220 nm Si layer; (b) five steps of ion implantation; (c) open the Ge window; (d) growth and smooth of germanium layer; (e) M1 finished; (f) M2 finished and annealing.
Fig. 3(a) shows the SEM of the waveguide, the width of the waveguide is 448 nm, which is within the process tolerance range. Fig. 3(b) displays the whole SEM of the grating, while Fig. 3(c) shows the enlarged view with a period and duty cycle of 486 nm and almost 0.5, respectively. Fig. 4 shows FIB view of the complete morphology of the device after finishing all preparation steps, and the depths of the Ge layer is 365.2 nm.
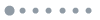
Figure 3.(a) SEM image of waveguide; (b) overall SEM plot of a grating; (c) SEM image of further enlarge of grating.
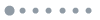
Figure 4.(Color online) Focused ion beam (FIB) view of the device’s structure.
Device performance parameters
Static photoresponse included I−V, responsivity, gain
Fig. 5 illustrates I−V curves of the device at 1310 nm with the input power between −10 to −25 dBm measured at room temperature. The APD breakdown voltage was defined when the current reaches 100 μA[22, 42]. At −21 V, the corresponding dark current is 38.6 μA, due to the source meter unit, so the breakdown voltage |VBD | > −21 V. In the dark I−V curves, the value of the initial dark current is unstable, which is mainly because the DC measurement system leakage current and additional parasitic capacitance. The value goes up from −0.7 to −8.8 V. At −8.8 V, the corresponding dark current is 1.12 nA. At low bias voltage, the dark current is low, because the electric field is mostly generated in silicon layer, so the dak current mainly comes from Shockley−Read−Hall generation and tunning[17, 43]. Subsequently, it further increases with voltage from −8.7 to −18 V. There are many factors that cause the dark current to rise. In this case, it is mainly the germanium−silicon interfacial electric field that plays a major role. As the voltage continues to increase, the dark current rapid rises, mainly because of the multiplication noise in APDs. The lattice mismatch between the germanium and silicon interface is approximately 4.2, which leads to numerous mismatches and threaded dislocation arrays, resulting in relatively high dark currents. Changes were made in the geometrical shape[18], growth temperature, and annealing conditions of Ge to enhance epitaxial growth[44, 45].
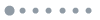
Figure 5.(Color online) Measured the dark and photo current I−V curves with input optical powers varying.
The photocurrent was experimental at the bias voltage ranging from 0 to −21 V. The measured photocurrent of the APDs is about 8.6 nA at 0 V and goes up quickly to 1.13 μA at −2.4 V with the input optical powers of −10 dBm. The germanium−silicon interface potential difference needs to be overcome[46, 47]. The photodetector increases with the voltage goes up, mainly because of the more carrier collection efficiency and avalanche multiplication. The photocurrent is 0.10 mA at −11.9 V, it shows further increase, this process is accompanied by the depletion of germanium region. At a voltage of 11.9 V, the photodetector registers a current of 0.1 mA, and it continues to increase. This process is associated with the depletion of the germanium region. When the voltage is much larger than −12 V, the photodetector increases result from the enhanced avalanche multiplication. When photodetector current reaches 100 μA, the bias voltage is 11.9, 15.3, 20.7, >21 V for the input optical powers of −10, −15, −20, −25 dBm, respectively. As the optical power increases, the photocurrent increases.
Fig. 6 shows the responsivity and gain against the reverse bias for four different input optical powers. The responsivity is the ratio of photocurrent (light current−dark current) to input power. The input optical power is the power in the APD. The multiplication gain is recognized as the ratio of the multiplied photocurrent R(V) to the primary photocurrent R0. The formula is G = R(V)/R0. The responsivity increases with the bias voltage increases. In the voltage range from 0 to −10 V, the responsivity remains below 0.5 A/W, a larger energy barrier needs to be overcome compared to pure silicon detectors, thus limiting the collection of carriers[43]. The growth quality of germanium epitaxy in Fig. 4, affects not only the electric field in the germanium region but also Ge-on-Si interface. At the heterogeneous interface of the germanium and silicon layers, the two types of atoms diffuse into each other, leading to a significantly reduced light absorption coefficient and hence low responsivity. The unit-gain responsivity is 0.5 A/W at −10 V, and the gain is assumed to be 1. As the voltage increases, the responsivity increases rapidly due to the avalanche effect. For instance, at −15 V, the responsivity is 2.98, 3.11, 3.01, 2.91 A/W for input optical powers of −10, −15, −20, −25 dBm, respectively. At −21 V, the responsivity increases to 4.84, 5.84, 7.62, 8.76 A/W at the optical power of −10, −15, −20, −25 dBm, respectively. Accordingly, as the bias voltage increases, the gain increases too in Fig. 6(b). For example, at −21 V, the gain is 9.68, 11.68, 15.24, 17.52 with the input optical power of −10, −15, −20, −25 dBm, respectively.
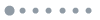
Figure 6.(Color online) (a) Calculated responsivity with the input optical power of −10, −15, −20, −25 dBm; (b) calculated multiplication gain with the input optical power of −10, −15, −20, −25 dBm.
Small-signal characteristics
To calculate the 3-dB bandwidth of the current Ge-on-Si APDs, the experimental setup for bandwidth characterization closely resembles the one described in paper[48]. The measurements circumstance is the same with the static measurements. Figs. 7(a)−7(c) depict the experimental frequency responses at varying bias voltages with the optical power of −10, −15, −20 dBm, respectively. Fig. 7(d) illustrates how the device's bandwidth changes at voltages ranging from −18 to −21 V and under three different incident optical power levels. At bias voltages between 0 and 18 V, the responsivity and gain are relatively low, because the built-in electric field is low, so electron−hole pairs cannot be effectively collected. Due to the low value, these results are not displayed in Fig. 7 at bias voltages between 0 and 18 V. As the voltage increases, the bandwidth also increases. However, at this point, the germanium absorption layer has not been fully depleted, leading to a longer transit time due to slow carrier diffusion. The bandwidth reaches its maximum at 20 V, as depicted in Fig. 7(d). As further increase in voltage, the 3-dB bandwidth decreases due to the accumulation of avalanche effects. At this stage, the time at which avalanche is initiated becomes a significant factor influencing the bandwidth[32, 43, 49, 50]. As the optical power decreases, for example, at −20 dBm, the observed peak enhancement in the frequency response, which is attributed to the LC resonance effect in avalanche region. This effect is the cause of the peak enhancement in the frequency response[33, 51, 21]. Furthermore, under high bias voltage, the peak is further amplified.
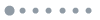
Figure 7.(Color online) Frequency responses with bias voltages varying from 18 to 21 V, (a) the optical power of −10 dBm, (b) the optical power of −15 dBm, (c) the optical power of −20 dBm, (d) measured 3 dB bandwidth under different optical powers at the bias voltage varying from 10 to 21 V from small signal measurements.
Fig. 8 shows the equivalent circuit of the present waveguide SCAM APD. In this circuit, the RC circuit is used to model the transit time of the absorber. An LC-circuit (LA and CA) is used for the avalanche region. The pad is the impedance of the APD with the parasitic effects, including the inductance Lp, the capacitance Cp, and the resistance Rp. At high bias voltages, avalanche effect is more obvious than the capacitance in absorber region, so the peak value becomes larger, at a certain frequency, the impedance of the APD has a maximal value. We use "peak value" to demonstrate the effect, the peak value is a positive value above the line of normalized S21 = 0.
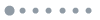
Figure 8.(Color online) The equivalent circuit of the present waveguide SCAM APD.
Fig. 9 is the setup for eye-diagram measurements, an arbitrary waveform generator (AWG) used to give a test signal, the high-speed oscilloscope tests the state of the device. The light from a 1310 nm laser passed through PC1 and was then modulated by a Mach–Zehnder modulator (MZM). The modulated light was sent into the unpackaged device under test (DUT) via PC2. A bias-tee was also employed in this setup. We used an erbium-doped fiber amplifier (EDFA) and a tunable filter to boost the signal then it reached into the APD. A sampling oscilloscope captures the output electrical signal. Fig. 10 displays the eye diagrams at 10 and 20 Gb/s at −21 V. The signal-to-noise ratio (SNR) decreases at higher data rates due to vertical eye closure.
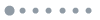
Figure 9.(Color online) Schematic of the experimental setup for the measurement of eye diagrams. The gray and red lines represent the optical and electrical paths, respectively.
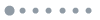
Figure 10.Experimental eye diagrams of the device at the bias voltage of 21 V. (a) At a 20 Gbps with the input optical power of −9 dBm; (b) at a 10 Gbps with input optical power of −9 dBm.
Discussion
Table 1 gives a benchmarking table of Ge-on-Si APDs, we compared the performance of the surface illuminated type device and the waveguide coupled device, meanwhile, the wavelength bands of 1310 and 1550 nm are shown in the table. In Table 1, compared with surface-illuminated APDs, the waveguide-coupled APDs have higher 3-dB bandwidths and more prominent responsivity[40]. Some waveguide-type APDs were with vertical SACM structures and thus require complicated fabrication processes. While, since there is no need for the silicon epitaxy growth and the metal contacts on the Ge layer, the lateral SACM APD structures have an easier fabrication process[30, 32]. This makes it very promising for integrating other functional devices (modulators, filters, etc.). The experiment result of present APD shows that at −21 V, the dark current measures approximately 38.6 μA, indicating the breakdown voltage for APD operation is greater than −21 V. The results show that in multiplication region the electrical field is weak, so a larger voltage is needed to induce avalanche or design a proper charge layer used technology computer aided design (TCAD). Furthermore, the APD shows a primary responsivity of 0.5 A/W, it is mainly caused by germanium epitaxy and metal absorption. We compared the peak value, in our work, the value is larger than 6, which is much larger than that is reported in article[33]. In actual production for APD device, the relationship between the bandwidth and eye diagram should be considered comprehensively[33].

Table 1. Benchmarking table of the waveguide Ge-on-Si APDs.
Table 1. Benchmarking table of the waveguide Ge-on-Si APDs.
Type | PN type | Λ(nm) | Id(nA) | R0(A/W) | Bandwidth (GHz) | Peakvalue | Year |
---|
Note: “/” indicates that the article does not mention this parameter. | Surface illuminated | Vertical SACM | 1550 | 30 | 0.30 | 8.0 | / | 2012[52] | Waveguide | Lateral PIN | 1550 | 600 | 0.49 | 33 | / | 2020[43] | Waveguide | Vertical PIN | 1550 | 20 | 0.8 | 21.0 | / | 2021[44] | Waveguide | Lateral SACM | 1310 | ~30 | 0.65 | 22.7 | / | 2021[30] | Waveguide | Vertical SACM | 1310 | 1 | 0.75 | 29.5 | / | 2021[53] | Waveguide | Lateral RT | 1310 | ~100 | 0.93 | 48.0 | / | 2022[32] | Waveguide | Vertical | 1550 | 35 | 0.81 | >75 | <3 | 2023[33] | Waveguide | Lateral SACM | 1310 | 38.6 | 0.5 | 15 | >6 | This work |
|
Conclusion
A waveguide-integrated lateral SACM Ge-on-Si APD using simplified fabrication processes with CMOS technology was studied. The device exhibits a primary responsivity of approximately 0.5 A/W at −10 V at O-band. Due to the source meter unit's limitations, at −21 V, the dark current is approximately 38.6 μA, suggesting that the APD's breakdown voltage exceeds −21 V. The discussion above details why the device shows a high breakdown voltage at the same dark current level. Concurrently, the device's 3-dB bandwidth is 15 GHz, achieved with the optical power of −15 dBm and a gain of 11.68. The 3-dB bandwidth curve shows that with low optical power, the peak bandwidth increases with rising voltage, which is more obvious at a higher voltage. This may lead to an increase in bandwidth. Finally, we performed the eye diagram test. The results indicate that at higher data rates, the SNR decreases due to vertical eye closure.