1. Introduction
High-power fiber lasers[1] have garnered significant attention in the fields of laser processing[2], laser weaponry[3], and other related domains due to their inherent advantages, such as superior beam quality[4], exceptional conversion efficiency[5], compact size[6], and user-friendly operation[7]. In recent years, high-energy short-range lasers have demonstrated their capability to effectively intercept tactical targets[8] within a limited range[9], rendering them highly destructive for impacted facilities. Consequently, the implementation of efficient safeguards against high-energy laser irradiation is increasingly imperative. Currently, laser protection technology primarily involves lens, window, and detector protection through optical thin films[10–12] and surface protection via metal or ceramic sprayed coatings[13–15]. The two protection types have very distinct application fields and protective effects. Using physical vapor deposition technology, which can control the thickness error within 2%[10], optical thin films can be applied to precision optical components with high laser damage resistance. For example, by adjusting the internal electric field distribution of each material, Nasiri et al. designed and manufactured all-dielectric and metal–dielectric mirrors with high laser-induced damage threshold (LIDT) for a continuous wave at 10.6 µm[11]. Instead, metal or ceramic sprayed coatings are surface treatment methods made of granular metal materials such as Al, silver, and gold powder, and ceramic materials such as , , , etc.[12], which act on large or shaped workpieces in heat- and radiation-resistant scenarios. They are primary applicable strategies for coarse surfaces of military equipment such as aircraft[13]. The substrate treated with spray coatings always shows a lower temperature rise effect on the back side. For example, Xue et al. used a coating made of and BN powders with a reflectance of 78.8% at 1064 nm, and the back temperature was 186°C when the laser power density of was continuously irradiated for 10 s[14]. However, further improvement of reflectivity is expected to obtain better antilaser coatings[15]. The current challenge lies in the application of laser-protective optical thin-film technology effectively applied to sprayed coatings, aiming to achieve enhanced reflectivity and resistance against laser damage on equipment surfaces.
Because of the limited hard damage range, laser weapons are usually used in close combat scenes. Visible light stealth[13,16,17] suitable for close combat is extremely important, which is usually achieved by camouflage technologies, such as multiband compatible stealth by employing Al powder and modified hybrid filler in an IRS coating[13] and whole-infrared-band camouflage with dual-band radiative heat dissipation by constructing seven-layer thin film[16]. The color of the coating primarily originates from the filler, whereas the film achieves controllable color by manipulating its structure. Introducing a Fabry–Perot (FP) cavity on the Al foil, Xiong et al. adjusted the parameters of the dielectric layer using different oxidation time and voltages to achieve a variety of colors for visible light stealth[17]. According to the characteristics of short-range laser protection, the research on thin films that possess both visible light stealth and excellent laser protection performance represents a crucial direction for development in the field of laser protection.
In this work, we propose a composite functional thin-film structure featuring compatibility with visible light stealth and laser protection, which is achieved by constructing an enhanced reflection asymmetric FP cavity. Consisting of a metal mirror layer, high-low index dielectrics, and an ultrathin metal layer, the structure can achieve higher reflectivity of laser wavelength while providing multidimensional stealth control mechanisms for achieving colors with varying hues and saturation to cater to diverse environmental requirements. The analysis focused on the synergistic relationship between background color and central wavelength reflectance, utilizing a 1064 nm laser. Finally, six distinct colors from blue to red were designed and prepared with a reflectance of more than 95% at the 1064 nm laser wavelength. The prepared thin films can withstand power density continuous irradiation for 60 s without being destroyed. Moreover, the symmetric thin-film structure applied to laser protective coatings, which has spectral consistency on both sides of the thin film, is proposed and realized. The blue coating is prepared and sprayed on the models to demonstrate the actual effect. This method verifies the feasibility of the functional thin film from theory to practical application.
Sign up for Chinese Optics Letters TOC Get the latest issue of Advanced Photonics delivered right to you!Sign up now
2. Simulation and Experiments
The evolution of thin-film structure in this paper is shown in Fig. 1(a). The traditional asymmetric FP cavity structure denoted as , consists of three layers: a metal layer, a dielectric layer, and an ultrathin metal absorbing layer. It exhibits color variation as the thickness of the dielectric changes. The metal–dielectric enhanced reflective film, as shows, can achieve high reflectance at the center wavelength within a few layers by periodically stacking high and low refractive index dielectric materials on a metal layer. Based on the above structures, we proposed an enhanced reflection type asymmetric FP cavity structure for laser protection thin film with compatible visible light stealth, which is depicted as . Due to the thickness of the initial metal layer grown on the substrate exceeding the skin depth of incident light, there is negligible electromagnetic field propagation at the interface between the substrate and the metal. As a result, subsequent layer growth is equivalent to occurrence on the base of the metal. The optical constants of the materials used in the experiment at the 400–1100 nm band are listed in Fig. 1(b). It should be noted that and exhibit zero absorption coefficients within this wavelength range; hence, they are not depicted. Figure 1(c) presents information on the melting point and appropriate deposition rate for each material. Although being in the innermost part of the film structure, Al possesses a much lower melting point compared to other materials. This can potentially lead to film failure under high-power fiber lasers and must be taken into consideration during film design and preparation.
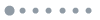
Figure 1.Structure and parameters of thin films. (a) Diagram of the thin-film structure; (b) optical constants of the materials; (c) melting points and deposition rates.
The spectral characteristics of structure and are identical at the central wavelength, while other bands exhibit distinct absorption effects. By carefully selecting the thickness of the absorbing layer, the structure can achieve a high reflectivity for laser wavelength and maintain controllable spectral characteristics within the visible light range. In this study, the thin film employs Al, Nb, and for low refractive index material while for high. Al is more cost-effective and readily available with broadband high reflection compared to silver and gold. The high melting point, chemical stability, and appropriate optical constants of Nb make it suitable as an ultrathin-absorbing layer material exposed to the atmospheric environment[18]. Dielectric films composed of and possess high damage thresholds and a large refractive index contrast ratio. This makes it easier to achieve high reflectivity at the laser wavelength and provides effective laser protection[19]. A change in the optical thickness of dielectrics is illustrated in Fig. 2(a). The color variation of different structures is illustrated in Fig. 2(a), and the reflectance of the central wavelength under different colors is indicated by characters with a standard of no less than 90%. The vertical and horizontal axes represent the thicknesses of and , respectively, in quarter-wave optical thickness (QWOT) units of the central wavelength (1064 nm). On the left side of the vertical axis and below the horizontal axis, color variation is observed with changes in dielectric thickness when and are, respectively, substituted for the dielectric layer in structure , which are denoted as () and (). The first quadrant region corresponds to the color change observed when altering the thickness of dielectrics on the structure with period , which is referred to as (). The color exhibited by () and () undergoes a one-dimensional change as the thickness of the dielectric varies. On the other hand, () features two layers of different dielectrics, enabling a two-dimensional approach to color regulation. This allows for achieving a more diverse and intricate range of colors. The laser reflectance of () and () does not exceed 93%, while the reflectance of () can surpass 96%. This demonstrates that () effectively enhances laser protection capabilities. For color blocks with equal optical thicknesses in the direction of slope such as the red line in Fig. 2(a), similar colors appear but exhibit slightly different hues. However, the electric field intensity distribution of each film is obviously different, as shown in Figs. 2(b) and 2(c). The marking of the curve corresponds to the coordinates in Fig. 2(a). For example, (0.2, 1.8) indicates that the thicknesses of and layers are 0.2 and 1.8 QWOT, respectively. Figure 2(d) shows the maximum and Al layer surface electric field intensity of each film. Selecting the appropriate dielectric thickness can effectively reduce the electric field intensity inside the film and protect the vulnerable material.
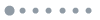
Figure 2.Color distribution and electric field regulation. (a) The color of the thin films and reflectance at the central wavelength (λ0 = 1064 nm) vary by changing the optical thickness of dielectrics. (b)–(c) The electric field intensity distribution changes with the thickness of each dielectric in the same total optical thickness. (d) The maximum and Al layer surface electric field intensity of each film.
The adjustment of the dielectrics’ thickness allows for the attainment of different colors, while a variation in color saturation is brought about by altering the thin Nb layer’s thickness. The schematic diagram of () is depicted in Fig. 3(a). Upon incidence of visible light and a 1064 nm laser, the film exhibits reflection properties that achieve both laser protection and visible-light stealth effect according to the designed target color. Under the assumption that the thickness of other materials remains constant, noticeable spectral variations can be observed by altering the thickness of the Nb layer. Figure 3(b) illustrates the spectra of () with Nb thicknesses ranging from 0 to 20 nm in increments of 5 nm. Using the 0 nm sample as a baseline reference, it is evident that introducing a thin layer of Nb induces significant changes in the spectrum. Typically, a 5 nm layer of Nb yields maximum color saturation. However, as the Nb layer thickness increases beyond this point, there is minimal alteration in film color while saturation gradually diminishes to achieve varying degrees of color intensity. In Fig. 3(c), the CIE 1931 coordinates corresponding to the colors of varying saturation in Fig. 3(b) are indicated, while the arrowed curve illustrates the trend of color coordinate change with increasing Nb layer thickness. Notably, by expanding the period of from to 2, theoretically incorporating an additional pair of high/low refractive index layers, the film can achieve higher central wavelength reflectance and consequently enhance laser protection effectiveness. The color of () is also investigated, as illustrated in Figs. 3(d)–3(f).
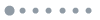
Figure 3.Characterizations on color saturation control. (a)–(c) Saturation of structure γ (n = 1) varies by changing the thickness of the Nb layer; (d)–(f) saturation of structure γ (n = 2) varies by changing the thickness of the Nb layer.
In contrast to (), () configuration exhibits multiple peaks and troughs within the visible-light spectrum, facilitating the attainment of mixed colors. By transitioning from a two-dimensional to a four-dimensional color space, () enables the achievement of colors that are challenging with ().
Finally, a set of six color samples ranging from blue to red was prepared in the experiment, as Fig. 4 shows, and the thickness parameters of each sample are shown in Table 1. The fan-shaped sample consisted of Al foil with a wrinkled surface, which is utilized to exhibit the appearance of films with rough surfaces in practical applications. On the other hand, the circular sample comprised a BK7 base and was employed for spectral measurement and laser protection testing purposes. The chromaticity coordinates for each sample and the target value of the design are depicted in Fig. 4(b). Among these samples, sample 1 () represents a film system structure consisting of () while sample 3 () corresponds to (). Further testing and analysis have been conducted on both and .

Table 1. Parameters of Different Samples
Table 1. Parameters of Different Samples
| 1 | 2 | 3 | 4 | 5 | 6 |
---|
Al (nm) | 100 | 100 | 100 | 100 | 100 | 100 | SiO2 (nm) | 91 | 193 | 222 | 178 | 243 | 272 | Ta2O5 (nm) | 152 | 80 | 56 | 117 | 84 | 79 | SiO2 (nm) | | 162 | 244 | | | 252 | Ta2O5 (nm) | | 143 | 94 | | | 109 | Nb (nm) | 5 | 5 | 5 | 6 | 10 | 10 |
|
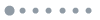
Figure 4.Preparation of different samples. (a) Samples on Al foil and BK7 substrates; (b) chromaticity coordinates of samples; (c) measurement and design spectra of S1; (d) measurement and design spectra of S3; electric field distributions of (e) S1 and (f) S3 at the wavelength of 1064 nm.
With more than 95%, the reflectance of and at the wavelength of 1064 nm is higher than that of a reference single-layer Al with a thickness of 100 nm, as shown in Figs. 4(c) and 4(d). Furthermore, the design spectra match well with the measurement spectra. The slight spectral redshift is attributed to the thickness error in film preparation and the measurement deviation. As shown in Figs. 4(e) and 4(f), both and effectively reduce the surface electric field intensity of Al from an initial value of to values of and , respectively, thereby providing enhanced protection for the Al layer, which possesses the lowest melting point.
In practical applications, devices are typically damaged when the protected substrate surface is irradiated after penetrating the protective films or coatings. To simulate real-world scenarios, our testing scheme deviates from measuring the laser-induced damage threshold and instead employs a method involving 1064 nm continuous laser breakdown of films. The measurement data includes laser power density, irradiation time, and spot ablation size. As depicted in Fig. 5, the expanded and collimated 1064 nm laser beam is split into two parts using a beam-splitting mirror: one with low energy and another with high. The low-energy beam is utilized to measure spot size and power density, while the high-energy beam impacts the BK7 sample surface. The sample is placed on a platform with a hollowed-out center. When a breakdown occurs, an energy meter positioned behind the sample platform detects the received energy. Our test utilizes an adjustable 0–20 W 1064 nm continuous fiber laser with a focused spot diameter of 0.8 mm, capable of reaching maximum power density of up to .
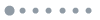
Figure 5.Schematic diagram of a laser testing device.
The experiment involved measuring the time taken to break down the film by irradiating the sample with varying power densities of the laser. Following fitting, is defined as the unbroken power density after 60 s of irradiation, while is the instantaneous broken power density. Subsequently, starting from 0, the laser was incrementally increased to at a rate of for pretreatment purposes[20]. Then, under different power densities, the breakdown probability after 60 s of irradiation was measured and recorded as and for points with probabilities of 0% and 100%, respectively. Figure 6(a) illustrates the laser irradiation test data of the single-layer 100 nm Al sample along with the fitted trend line. The measurement findings indicate that under irradiation of , the single-layer Al remains intact for a duration of 60 s and can be enhanced to after undergoing laser pretreatment. However, even after laser pretreatment, when the laser power density reaches , there is still a probability exceeding 80% for breakdown occurrence in single-layer Al. Correspondingly, the laser irradiation test curve of is shown in Fig. 6(b), with () at and () increasing to . It should be noted that the breakdown probability of the at is reduced to less than 60% after pretreatment. The laser pretreatment process enhances the lower limit of laser protection ability for single-layer Al by effectively reducing surface impurities and minimizing absorption. However, when exposed to higher power density, single-layer Al undergoes melting and expansion, leading to surface flatness damage, further amplifying absorption, and creating a positive feedback loop. In contrast, since is stacked with dielectric layers that possess a certain porosity, it allows room for Al expansion without affecting the film system’s surface topography, thereby significantly improving its laser protection ability.
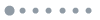
Figure 6.Laser irradiation test results. (a) Test curve of laser irradiation on single-layer Al; (b) test curve of laser irradiation on S1; (c) single-layer Al micrograph of the front after irradiation; (d) S1 micrograph of the front after irradiation; (e) single-layer Al micrograph of the back after irradiation; (f) S1 micrograph of the back after irradiation.
The micrographs of the front and back of the single-layer Al sample and after laser irradiation are shown in Figs. 6(c)–6(f). Among them, the large hole represents test points without laser pretreatment, exhibiting varying degrees of ring-shaped irradiation traces or crack damage. The small hole represents a test point after laser pretreatment, demonstrating that laser pretreatment effectively reduces the damaged area, particularly in the case of . Due to the limitations of the instrument, no obvious damage was captured by the eyes or optical microscope even after irradiation of for 60 s at a maximum power density.
In the series of scanning electron microscope (SEM) images, Fig. 7(a) depicts the surface morphology of crack damage on the large hole shown in Fig. 6(d), exhibiting black patches indicative of impurities or ablative marks near the crack. Conversely, Fig. 7(b) illustrates specific characteristics of the small hole of in Fig. 6(d), displaying a regular ablative area with fewer surrounding black patches. Through comparison, it is evident that laser pretreatment effectively reduces the damaged area and yields more regular morphological features. Furthermore, Fig. 7(c) showcases ablation marks on after laser pretreatment without breakdown, representing significantly smaller irradiation traces. Additionally, Figs. 7(e) and 7(f) separately depict sections of before and after irradiation for 60 s, with the highest power density during the experiment. It can be observed that the initial damage occurs in Al, despite the smooth surface, as shown in Fig. 7(d). Subsequently, molten Al infiltrates into the pores of the dielectric layers without compromising surface flatness, thereby facilitating laser protection.
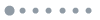
Figure 7.Series of SEM images. (a) Surface morphology of S1 exhibiting crack damage; (b) surface morphology of S1 exhibiting an ablative hole. (c) Surface morphology of S1 exhibiting a trace of irradiation under an unbroken test. (d) Cross section of 100 nm single-layer Al; (e) cross section of S3; (f) cross section of S3 irradiated with the highest power density laser for 60 s.
The conversion from thin film to coating is the key point to the practical application of laser-protective films on irregular surfaces. To realize this conversion, the symmetric thin-film structure based on an asymmetric FP cavity is proposed. This structure ensures the spectral consistency of the microreflectors formed after the film is fragmented. Regardless of which side the light incident originates from during the irradiation process, the same spectral modulation effect can be attained.
The schematic diagram of the film layer plated on the BK7 substrate after achieving structure symmetry is shown in Fig. 8(a). The front light enters the film layer from the air side and ultimately obtains its spectrum on the air side. On the other hand, the back light passes through a 2 mm thick BK7 base when an incident from the air before reaching the surface of the film layer. The reflected light is then received through the substrate into the air, with additional Fresnel reflection occurring at BK7, resulting in certain differences in both front side and back side spectra. In Fig. 8(b), blue and red lines, respectively, represent the front reflection spectrum and back reflection spectrum. The dashed line represents the simulation design curve while the solid line represents the sample test curve. Figure 8(c) illustrates two sides of colors of the symmetric structure under the BK7 base, where letters F and B denote the front and back sides of the sample, respectively. Although there is a minimal color difference between both sides, the back side exhibits two different images due to the Fresnel reflection effect. Figure 8(d) displays an SEM cross-sectional image showing good symmetry on both sides of Al, which are symmetrical about the Al layer itself. Based on the symmetric structure, film stripping material is plated between BK7 and Nb layer near BK7 to obtain microreflectors that can be further converted into coatings, as Fig. 8(e) shows. By spraying coatings on car models, it can be observed that the base color has minimal impact on the sprayed color shown in Fig. 8(f), indicating that the coating material can be applied to surfaces of any color and shape.
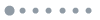
Figure 8.Verification and application of thin films with symmetrical structure. (a) Schematic diagram of symmetrical S1 structure on the base; (b) front side and back side spectra of symmetrical S1; (c) colors of front side and back side. (d) cross section of symmetrical S1; (e) collection of S1 microreflectors; (f) coating spraying effect.
3. Conclusion
In summary, the enhanced reflection asymmetric FP cavity structure combines the high reflection from the metal–dielectric enhanced structure and color control of the traditional asymmetric FP cavity. The color control is accompanied by an optimization of the electric field distribution within the film, considering the material characteristics. To replicate the actual application environment, the laser test scheme for breakdown measurement is employed in this experiment. Compared to a 100 nm single-layer Al reference, the power density of experiencing breakdown after 60 s of irradiation increased from to . Additionally, the power density with zero probability of breakdown after 60 s of continuous irradiation following laser pretreatment increased from to . Moreover, the laser pretreatment process is capable of effectively reducing the damaged area. Finally, the symmetric structure thin film was designed and prepared with a good agreement between the spectra obtained from both the front and back sides. Due to the color adjustability of the thin film and coating, this structure can be applied to laser protection scenes in various background color environments and provides a new method for the future laser protection field.