Introduction
In recent years, an increasing number of organic pollutants, such as antibiotics[1, 2], phenols[3, 4], and other organic pollutants, have been widely present in water bodies, which can be easily discharged into the aquatic environment through domestic and industrial effluents and cannot be eliminated by conventional water treatment methods[5, 6]. Most of the emerging pollutants are usually considered to be persistent and carcinogenic[7, 8], and long-term exposure may adversely affect human and animal health, posing a great threat to the ecosystem and human health[9−11]. Therefore, there is an urgent need for research to identify and develop a sustainable purification method to remediate these pollutants. Therefore how to remove organic pollutants from water is very important for environmental remediation and protection of the environment. In the past decades, a variety of treatment methods have been investigated for the removal of emerging pollutants, including membrane filtration[12], adsorption[13], flocculation precipitation[13], ion exchange[14], electrochemical methods[15], and ozone oxidation techniques[16]. High energy consumption and the tendency to cause secondary pollution are common problems in these treatment methods. Semiconductor-based photocatalytic technology has attracted much attention in the ecological remediation of emerging pollutants due to its unique features such as low cost, simple operating procedures, and high degradation potential under light irradiation[17−19].
The photocatalytic semiconductors can absorb light energy to generate photoelectrons and photogenerated holes[20, 21], and the holes have a strong oxidizing ability, which can generate numerous oxidizing active species in water to degrade organic pollutants, while the holes can also oxidize the pollutants directly, and the photocatalytic technology does not need to use external chemicals in water treatment, so it is a widely recognized green and economic water treatment technology[22−24]. However, the photogenerated electrons and photogenerated holes of photocatalytic semiconductors are not well separated after generation, and most of them are easy to compound after generation, which greatly limits the practical application of photocatalysts[25−27]. Therefore how to reduce the problem of photocatalytic semiconductor photogenerated carriers prone to compounding is an important problem[28−30]. The large accumulation of photogenerated carriers on the surface that cannot be consumed during the photocatalytic process may be the main reason for the high composite rate and low utilization of photogenerated carriers, and therefore the spatial separation of electrons and holes by photogenerated electron trapping is a possible strategy[31−33].
Perylene diimide (PDI) supramolecular semiconductor photocatalysts possess a wide range of light-absorbing abilities to effectively absorb visible light and produce oxidatively active species[34−36], but PDI faces the same problem of inefficient charge separation as other catalysis[37]. Therefore here we combine PDI with the non-homogeneous Fenton catalyst iron hydroxyl oxide (FeOOH) to obtain FeOOH@PDI, which enhances the photogenerated hole release from PDI by trapping the photogenerated electrons on the surface of PDI by Fe(Ⅲ) in FeOOH. The photocurrent and steady-state fluorescence spectra show that FeOOH has greatly enhanced the charge separation efficiency of PDI, and the complexation of photogenerated electrons and photogenerated holes is suppressed, while the surface photovoltage spectra also indicate that the combination of FeOOH with PDI greatly increases the photogenerated holes on the PDI surface. We combined FeOOH with Fenton technology to form a photo-Fenton system with FeOOH@PDI as the catalyst, in which the degradation of organic pollutants was greatly enhanced. Species capture experiments demonstrated that the released photogenerated cavities can effectively degrade organic pollutants to enhance the degradation rate of organic pollutants. In this work, an effective material was constructed to enhance the interfacial charge separation of PDI, which effectively overcame the problem of easy compounding of photogenerated holes and photogenerated electrons in photocatalytic semiconductors by rapidly transferring the photogenerated charge on the surface of the semiconductor, and achieved rapid degradation of pollutants in water.
Experiment
Preparation of FeOOH
Weigh 329.6 mg FeCl3•6H2O and add it into 60 mL deionized aqueous solution, adjust pH = 4 with 1M NaOH, and stir for 30 min. It was also transferred to a 100 mL PTFE−lined hydrothermal reactor and heated at 80 °C for 6 h. After the reaction, the obtained solids are filtered, washed with deionized water 3 times, and finally freeze-dried.
Preparation of self-assembled PDI
Self-assembled PDI was synthesized according to the reported methods[38]. 3.5 mmol PTCDA, 18 g imidazole, and 28 mmol β-amino propionic acid were mixed under argon atmosphere with stirring at 100 °C for 4 h. After the reaction product was cooled naturally to room temperature, 100 mL ethanol and 300 mL HCl (2.0 M) were added and stirred overnight to obtain a dark red mixed solution. The mixture was washed with ultrapure water to neutral and dried under vacuum at 60 °C for 24 h to obtain bulk PDI. 300 mg synthesized bulk PDI was sonicated and dispersed in 109 mL water. Then, 453 mL TEA was added during stirring to ensure complete dissolution to form a dark red PDI stock solution. Afterward, 14.13 mL HCl (4.0 M) was added to the stock solution and stirred for 1 h. The obtained precipitate was washed with ultrapure water to neutral and dried under vacuum at 60 °C for 24 h. The final dark red product was the self-assembled PDI.
Preparation of FeOOH@PDI
The synthesis scheme of FeOOH@PDI is shown in Fig. 1. Weigh 32.96, 65.92, 98.88, 131.84 mg FeCl3•6H2O dispersed in 40 mL of deionized water, adjust the pH = 4, and weigh 90, 80, 70, 60 mg of self-assembled PDI dispersed in 20 mL of deionized water, PDI dispersion was added dropwise to the corresponding trivalent iron solution, stirred for 30 min. The PDI dispersion was added dropwise to the corresponding trivalent iron solution and stirred for 30 min. It was also transferred to a 100 mL PTFE-lined hydrothermal reactor and hydrothermally heated at 80 °C for 6 h. At the end of the reaction, the obtained solids were filtered, washed with deionized water 3 times, freeze-dried, and named 10%, 20%, 30%, and 40% FeOOH@PDI, respectively.
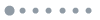
Figure 1.(Color online) Schematic diagram of the composite route at FeOOH@PDI.
Experimental condition
The experiments were carried out in 60 mL quartz tubes with a volume of 50 mL, and the 20 ppm organic pollutant, 400 mg/L of catalyst was added in each set of degradation experiments. In the anti-interference experiments, The amount of contaminant and catalyst was kept constant, and 100 ppm of interferon was added to each set of anti-infection experiments. In the cycling experiments, the catalyst was filtered and washed with deionized water after each cycle, and the washed catalyst was added directly to the next cycling experiment.
Analytical methods
High-performance liquid chromatography (HPLC) was used to determine the residual content of organic contaminants. X-ray photoelectron spectroscopy (XPS) is used to characterize elemental valence states and changes in chemical bonding of FeOOH@PDI surfaces. The transmission electron microscope-energy dispersive spectrometer (TEM-EDS) was used to characterize the morphology of FeOOH@PDI and the elemental species. The scanning electron microscope (SEM) is used to observe the surface morphology of materials. Fourier transform infrared spectrometer spectroscopy (FT−IR) is used to characterize the chemical bonding and functional groups of materials. X-ray diffractometer (XRD) is used to characterize crystallinity changes in materials.
Results and discussion
The structure analysis of FeOOH@PDI
As shown in Fig. 2(a), it can be seen in the XRD pattern that the peak of PDI decreases significantly after the combination of FeOOH and PDI, indicating that the surface of PDI is combined with the amorphous FeOOH, and the shielding effect of the surface FeOOH on the X-ray makes the peak strength of PDI gradually decrease with the increase of FeOOH. It can also be seen from the Zeta potential that there is a big difference between the surface Zeta potential of PDI and FeOOH. PDI has a negative potential due to its dense ring structure and the deprotonation of the carboxyl group on the surface, while for FeOOH, the surface hydroxyl group has a positive surface potential. Therefore, the surface potential of the two materials can be neutralized after combining, so that the Zeta potential of FeOOH@PDI lies between the two materials (Fig. 2(b)). It can be seen in Fig. 2(c) infrared spectra that the synthesized FeOOH can indeed effectively combine with PDI and an obvious Fe−O and Fe−O−H bond is found in 20% of the FeOOH@PDI infrared spectra[39].
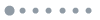
Figure 2.(Color online) (a) FeOOH@PDI, XRD patterns of PDI and FeOOH. (b) Zeta potential for FeOOH, PDI, FeOOH@PDI. (c) 20% FeOOH@PDI, PDI, and FeOOH infrared spectra.
In the TEM and EDS spectra, it can be seen that Fe elements are uniformly distributed on the surface of PDI, and it can be seen in Fig. 3(a) that FeOOH is effectively embedded in the PDI nanoribbons. And in the TEM-EDS spectra Figs. 3(b)−3(f), it can be seen that iron elements are uniformly distributed on the PDI. Meanwhile, Fig. 3(g) shows that FeOOH and PDI are not only physically bonded, but there is also a certain binding force between FeOOH and PDI, which can be seen in the C 1s peaks of PDI. After FeOOH is loaded, the C=O and O=C−O binding energies of PDI are increased from 287.80 and 289.00 eV to 287.83 and 289.21 eV, respectively. which indicates that there is an obvious tendency of electron transfer in these two groups, which may be due to the iron ions on the surface of FeOOH directly binding to the carboxyl group of PDI resulting in the tendency of electron transfer on the carboxyl group of PDI. Combined with the above analysis, FeOOH and PDI were successfully combined to obtain FeOOH@PDI.
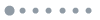
Figure 3.(Color online) TEM spectrum of (a) 20% FeOOH@PDI and TEM-EDS image of (b)−(f) 20% FeOOH@PDI. (g) PDI and 20% FeOOH@PDI C 1s spectrum.
In the SEM patterns, it can be seen that FeOOH presents irregular particles, and the PDI presents nano-strips with relatively smooth surfaces (Figs. 4(a) and 4(b)). The size of FeOOH nanoparticles is much smaller than that of PDI, and it can be seen that FeOOH is loaded on the surface of PDI. It can seen in Fig. 4(c) that the FeOOH loaded on the PDI surface made the smooth PDI nanoribbons rough.
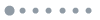
Figure 4.SEM images of (a) FeOOH, (b) PDI, and (c) 20% FeOOH@PDI.
The degradation properties of FeOOH@PDI
To investigate the photo-Fenton catalytic performance of FeOOH@PDI, we used FeOOH@PDI in the experiment of photo-Fenton degradation of organic pollutants. A period of dark adsorption was still performed at the beginning of the experiment. Meanwhile, 20 μL of H2O2 (30%) was added to the reaction system to start the photo-Fenton reaction at the switch. From the degradation results of bisphenol A (BPA) in Figs. 5(a) and 5(b), it can be seen that the photo-Fenton activity of FeOOH@PDI is significantly higher than that of pure PDI, which is mainly due to the increase of FeOOH which enables the effective activation of H2O2 to produce hydroxyl radicals (•OH), and the reaction rate constants show that the activity of 20% FeOOH@PDI is the highest. The activity of 20% FeOOH@PDI is the highest, and the degradation rate of BPA reaches 0.112 min−1. From Figs. 5(c) and 5(d), we can see that the reaction activity of different materials under the conditions of photo-Fenton reaction also differs greatly, and pure FeOOH can activate the hydrogen peroxide for the degradation of BPA, and there is no significant increase in the activation effect of FeOOH for hydrogen peroxide under the light, which indicates that light does not have a significant effect on the photo-Fenton reaction of FeOOH, and secondly, the degradation ability of photo-Fenton in the mixed system of 80% PDI and 20% FeOOH is better than FeOOH will be. However, the reaction rate of the 20% FeOOH@PDI photo-Fenton system is much larger than the above-mentioned photo-Fenton systems. This indicates that the combination of PDI and FeOOH is not an ordinary mixture and that there must be a close bond between the two materials to have better activity, it also indicates that the presence of PDI is the key to the enhancement of the activity. 20% FeOOH@PDI photo-Fenton activity is not only for BPA but also for the other organic pollutants that may be present in the water, For example, antibiotics such as ciprofloxacin (CPFX) oxytetracycline (OTC) and tetracycline (TC), etc. This indicates that the FeOOH@PDI system is also universal in its ability to degrade difficult emerging pollutants (Fig. 5(e)). The FeOOH@PDI system also has a good removal rate of total organic carbon for organic pollutants, in which the mineralization rate of phenol can reach 88.01%, in addition to other organic pollutants also has a good mineralization rate (Fig. 5(f)).
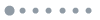
Figure 5.(Color online) (a) and (b) Degradation effects and degradation rate constants of BPA by PDI and FeOOH@PDI. (c) and (d) Degradation effects of different Fenton reaction modes on BPA and degradation rate constants. (e) 20% FeOOH@PDI degradation effect and degradation rate constant of different organic pollutants. (f) Mineralization rate of organic pollutants.
Anti-interference performance
To investigate the stability of the 20% FeOOH@PDI photo-Fenton system, several anions, cations, and humic acid (HA) are added into our constructed reaction system, respectively, to explore the effect of different components on the efficiency of organic pollutant removal (Fig. 6). We found that the effect of HPO42− on the removal of pollutants is relatively serious, it is because Fe(Ⅱ) and Fe(Ⅲ) in FeOOH may react with HPO42− to form FePO4, which will mask the active sites of the photo-Fenton to reduce the non-homogeneous Fenton activity. Humic acid (HA) and HCO3− also had a slight effect on the degradation effect, probably due to the bursting effect of HA and HCO3− on hydroxyl radicals and holes. In general, the 20% FeOOH@PDI photo-Fenton system is highly resistant to interference and has the potential for the removal of organic pollutants in real water.
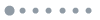
Figure 6.(Color online) Photo-Fenton degradation of 20 ppm BPA by 20% FeOOH@PDI under different ions and humic acid interference.
Charge transfer mechanism
In the photocurrent test, it can be seen that the photocurrent of PDI is significantly enhanced after the combination of FeOOH and PDI, in which the photocurrent of 20% FeOOH@PDI is significantly higher than that of the other materials Fig. 7(a), and the electrochemical impedance spectroscopy (ESI) test also indicates that the combination of FeOOH and PDI can effectively reduce the charge transfer resistance of the materials, which may provide favorable conditions for the interfacial electron transfer process Fig. 7(b). we found that the interfacial electron transfer enhancement may promote the charge separation efficiency of the material, which in turn reduces the compounding rate and releases the holes of PDI. The steady-state fluorescence (PL) Fig. 7(c) as well as the surface photovoltage spectrum (SPV) Fig. 7(d) indeed also proves this point of view, from the steady-state fluorescence spectra we can see that the fluorescence in FeOOH@PDI is much lower than that of the pure PDI, it indicates that the photogenerated carrier recombination of the PDI is significantly restrained in this process, while the surface photovoltage (SPV) of FeOOH@PDI is higher than that of pure PDI, which means that the photogenerated hole concentration on the surface of FeOOH@PDI is further improved. Although FeOOH can effectively promote PDI charge separation, the content of FeOOH is not the higher the better, too high the content of FeOOH will affect the light absorption of PDI. After testing, we found that 20% FeOOH@PDI has the best photoresponse and charge separation, this is also consistent with the results of degrading pollutants.
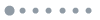
Figure 7.(Color online) (a) Transient photocurrent, (b) electrochemical impedance spectroscopy, (c) steady-state fluorescence (PL), and (d) surface photovoltage (SPV) of PDI and FeOOH@PDI.
Cycle stability test of FeOOH@PDI
As shown in Fig. 8(a), in the degradation stability test of FeOOH@PDI material, it was found that the degradation effect of the catalyst on BPA only decreased by 7.14% after five cycles (each reaction time of 30 min), which indicates that FeOOH@PDI is a stable and feasible photo-Fenton catalyst. In FeOOH@PDI, FeOOH is the active site center for activating hydroxyl radicals generated from hydrogen peroxide, and it is also the key to promoting the charge separation of PDI to accelerate the release of photogenerated holes. We measured the valence and ratio of Fe on the catalyst surface before and after the reaction (Fig. 8(b)), and the ratio of Fe(Ⅲ) : Fe(Ⅱ) decreased from 0.536 before to 0.491 after the reaction (the content of Fe(Ⅱ) increased after the reaction), which indicates that the photogenerated electrons generated by PDI photoexcitation can effectively reduce Fe(Ⅲ) on the surface of FeOOH during the reaction to generate Fe(Ⅱ). In conclusion, FeOOH@PDI can effectively solve the disadvantage that Fe(Ⅲ) in FeOOH cannot be converted to Fe(Ⅱ) quickly, which also enhances the catalyst's stability.
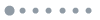
Figure 8.(Color online) (a) Cyclic stability of 20% FeOOH@PDI, (b) valence states, and proportions of Fe on FeOOH@PDI surface before and after the reaction.
Conclusion
In this work, the FeOOH@PDI catalyst was successfully synthesized and used in conjunction with Fenton technology to construct a photo-Fenton degradation system. In this system, organic pollutants were efficiently degraded, and the degradation rate of BPA reached 0.112 min−1, which was 20 times higher than that of the PDI system alone, and the system also had a wide range of degradation abilities for other phenolic organic pollutants and emerging pollutants such as antibiotics. This system also showed strong anti-interference performance under the interference of different ions and humic acids (HA). Mechanistic research shows that the photogenerated electrons generated on the surface of FeOOH@PDI after light excitation can be effectively captured by FeOOH, which promotes the charge separation of PDI and restrains the recombination of photogenerated electrons and photogenerated holes, and the release of holes is further promoted. It is more conducive to the degradation of organic pollutants. Fe(Ⅲ) on the surface of FeOOH was converted into Fe(Ⅱ) after capturing the photogenerated electrons, which provided abundant active sites required for the reaction and accelerated the activation of hydrogen peroxide. Moreover, the photo-Fenton system also has good recyclable performance, which still maintains a good degradation rate of pollutants during the recycling process. Our work suggests feasible strategies for enhancing the charge separation efficiency of photocatalytic semiconductors and potential viable solutions for the removal of emerging pollutants in water.