1. Introduction
Lasers emitting in the 3-µm wavelength region are of great significance for a number of applications. In medicine, such lasers can be utilized for precise surgery of soft or hard tissue with very low thermal damage[1]. This is enabled by the global absorption maximum of water at 2.94 µm, leading to penetration depths in tissue on the order of few micrometers. 3-µm radiation is also of utmost importance for gas sensing. The fundamental absorption lines of methane ()[2], water vapor (H2O), nitrous oxide ()[3], ammonium ()[4], and carbon monoxide (CO)[4] are located in this wavelength region. Thus, highly sensitive gas sensing can be achieved with 3-µm lasers. However, most of these applications lack directly emitting high-power continuous-wave (CW) laser sources. Thus, inefficient and complex nonlinear conversion schemes are often applied to generate 3-µm radiation[4,5]. In addition to the chemical formulas above, further applications could benefit from directly emitting lasers with improved operation parameters in the 3-µm range.
Using as the active ion in diode-pumped solid-state lasers is a promising approach for generating laser at 3 µm. This is owed to a potentially high-energy conversion efficiency, a compact and simple setup, and the possibility of choosing a suitable gain media for the desired application parameters. As shown in Fig. 1, the 3-µm laser emission in is based on the transition . As the radiative lifetime of the upper laser level () is much shorter than that of the lower laser level (), this transition should be self-terminating. However, this issue can be tackled by using high doping concentrations. These facilitate depopulation of the lower laser level via energy transfer upconversion (ETU) ( and ), and of the upper laser level ( and )[6]. Consequently, these non-radiative depopulation processes reduce the radiative lifetimes of both levels. However, the lower laser level is typically strongly affected by this process. In addition, the ETU transition from the lower laser level re-populates the upper laser level via a successive non-radiative decay . Consequently, high doping concentrations are favorable for creating stable population inversion and avoiding self-terminating laser operations. Moreover, the latter process enables the creation of more than one laser photon at with a pump photon at , allowing for slope efficiencies exceeding the Stokes limit of [7,8].
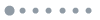
Figure 1.Simplified energy levels of the Er3+ ions and the energy transfer mechanism.
Fluoride and oxide materials are two kinds of promising gain materials of the laser in this region. Fluoride materials show lower phonon energy (), which will reduce the possible non-radiative multi-phonon relaxation of [9] and support higher laser efficiencies. [7] ([10]) and [11] ([9]) have achieved extremely high slope efficiencies () of 50% and 41%, respectively, while oxide materials such as (Er:YAP)[12] () and [13] () show slope efficiencies of 32% and 36%, respectively. However, oxide materials exhibit higher thermal conductivities. This means that the gain materials can tolerate a higher pump power, leading to higher laser powers. (crystal)[13], (ceramic)[14], Er:YAP (crystal)[12], and (ceramic slab)[15] have delivered output powers of 5.9 W, 6.7 W, 6.9 W, and 13.4 W, at room temperature. Cooling with liquid nitrogen (77 K), output power of 24 W[8] was realized with , while the highest output power among the fluoride lasers is by Er:YLF[16].
Sign up for Chinese Optics Letters TOC Get the latest issue of Advanced Photonics delivered right to you!Sign up now
Among the reported gain media, is one of the most promising candidates used to achieve high-power mid-infrared laser output. It shows a high thermal conductivity, even at high doping concentrations[17]. Here, diode single- and dual-end-pumped CW laser oscillator concepts are investigated with the aim of further power scaling. In a single-end-pumped resonator, a slope efficiency of 25.5% was realized with an output power of 10.1 W at an absorbed pump power of 41.9 W. By applying a dual-end-pumping scheme, we homogenized the thermal load in the gain material and achieved the highest output power of any room-temperature crystalline -doped gain crystal in the mid-infrared, so far. A laser output power of 14.1 W was achieved at a total absorbed pump power of 59.7 W. The corresponding slope efficiency amounted to 26% and the power stability is better than over one hour. To the best of our knowledge, this is the first -based, room-temperature, 3-µm CW crystalline laser with more than 10 W of output power.
2. Experimental Setup
The experimental setup is depicted in Fig. 2. Figures 2(a) and 2(b) show the single- and the dual-end-pumped resonator configurations, respectively. In both cases, the gain medium is a Er(7%, atomic fraction):Lu2O3 crystal grown by the heat exchanger method[18] and cooled to 10°C (prepared by Christian Kränkel). The input coupling mirror (IC) is concave with a radius of curvature of 10 m. It is anti-reflection (AR) coated for the range of the pump wavelength between 960 nm and 990 nm and high-reflection (HR) coated for the range of possible laser wavelengths between 2700 nm and 2900 nm. We used two different plane mirrors as output couplers (OCs) for the laser radiation. These are AR coated in the pump wavelength range and exhibit transmissions (T) of 3% and 5% for the laser wavelength. To avoid resonator losses via water vapor absorption, the laser cavity was set up to be as short as possible, leading to -long resonators. The coupling fiber of the 100-W pump diode has a core diameter of 105 µm and an NA of 0.22. The pump laser wavelength is stabilized at 976 nm with a full spectral width at half maximum of 0.4 nm. In the single-end-pumped scheme [Fig. 2(a)], the pump beam is collimated by lens L1 and then is focused into the laser crystal by lens L2. In the dual-end-pumped scheme [Fig. 2(b)], the collimated pump beam is separated by a polarizing beam splitter (PBS). The p-polarized (p-pol.) pump beam is focused into the gain medium from the forward direction by lens L2, and the s-polarized (s-pol.) light is applied from the backward direction by lens L3. Such a polarization beam separation design is also useful for protecting the laser diode from the damage of the residual pump light in the backward direction but should not affect the performance of the cubic crystal. In both schemes, the laser signal is separated from the residual pump by a 45° dichroic mirror (DM), which is AR coated for the pump and HR coated for the laser wavelength incident under this angle.
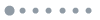
Figure 2.Laser schemes of (a) the single-end-pumped and (b) the dual-end-pumped Er:Lu2O3 lasers.
3. Laser Experiments and Results
3.1. Laser performance of water-cooled lasers
By placing the crystal, which is tightly wrapped in indium foil, in a water-cooled copper heat sink, we investigated power scaling of the single- and dual-end pumped resonators. First, the CW laser performance of the single-end-pumped laser was investigated with a coupling lens group, in which the focal lengths of the lenses L1 and L2 are 30 mm and 150 mm, respectively, yielding a coupling group of 30:150. For , the resonator allowed for a maximum of 6.0 W of laser output under 27.5 W of absorbed pump power. For the 5% OC mirror, 6.2 W was realized under 32.8 W of pump power (Table 1). The corresponding slope efficiencies amount to 24% and 21% using the OC mirrors of 3% and 5% transmission, respectively. Limited by the serious thermal load, a higher output power was not feasible by further enhancing the pumping power.

Table 1. Summary of the Results Obtained with Different Er:Lu2O3 Resonator Configurations
Table 1. Summary of the Results Obtained with Different Er:Lu2O3 Resonator Configurations
Cooling | Laser Scheme | Coupling Lens Group | Pump Direction | Polarization | Output Coupler Transmission (%) | Maximum Absorbed Pump Power (W) | Highest Output Power (W) | Slope Efficiency (%) |
---|
Water | Single-end-pumped scheme | 30:150 | Forward | Non-pol. | 3 | 27.5 | 6.0 | 24 | 5 | 32.8 | 6.2 | 21 | 35:200 | Forward | Non-pol. | 3 | 32.0 | 8.1 | 28 | 5 | 32.0 | 7.2 | 25 | Dual-end-pumped scheme | 30:150:150 | Forward & backward | / | 3 | 42.7 | 8.1 | 21 | 5 | 38.9 | 7.2 | 21 | Forward only | p-pol. | 3 | 26.0 | 5.2 | 22 | 5 | 26.0 | 4.4 | 20 | Backward only | s-pol. | 3 | 27.9 | 4.8 | 19 | 5 | 27.9 | 4.7 | 19 | 35:200:200 | Forward & backward | / | 3 | 43.6 | 10.1 | 25 | 5 | 33.5 | 7.3 | 25 | Forward only | p-pol. | 3 | 24.7 | 6.6 | 28 | 5 | 25.6 | 5.9 | 26 | Backward only | s-pol. | 3 | 26.8 | 5.3 | 22 | 5 | 27.9 | 5.0 | 21 | TEC | Single-end-pumped scheme | 35:200 | Forward | Non-pol. | 3 | 41.9 | 10.1 | 27 | 5 | 41.9 | 9 | 25 | Dual-end-pumped scheme | 35:200:200 | Forward & backward | / | 3 | 59.7 | 14.1 | 26 | 5 | 59.7 | 12 | 22 |
|
To improve the output power, the thermal intensity was reduced by enlarging the pump beam diameter to with a coupling lens group of 35:200. As shown in Fig. 3(a), slope efficiencies of 28% and 25% were achieved with OCs of 3% and 5% transmission, respectively. Under a maximum absorbed pump power of 33.3 W, output powers of 8.1 W and 7.2 W, respectively, were achieved in the configuration shown in Fig. 2(a). Thus, in the 35:200 configuration, the slope efficiencies were improved by a factor of 1.2. Theoretical calculations show that perfect mode matching requires an even larger pump diameter of , but we did not find improved results using a 30:200 coupling group for further enlarging the pump beam diameter.
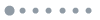
Figure 3.Laser characteristics of (a) the single-end-pumped and (b) the dual-end-pumped setups for different T by using 35:200 and 35:200:200 lens group, respectively.
The dual-end-pumping scheme can create a more homogenous distribution of the pump light and thus of the inversion in the crystal. Due to the long lifetime and significant population of the lower laser level, the -based 3-µm-laser can be treated as a three-level laser system. For such lasers, a more homogeneous distribution of the population inversion can reduce the reabsorption of the laser photons[19]. Moreover, a homogenous distribution of the pump light also homogenizes the thermal load in the gain area, enabling higher pump and thus output powers[20].
Using a 30:150:150 lens group (the focal lengths of the lenses L1, L2, and L3 are 35 mm, 200 mm, and 200 mm, respectively), we described the performance of the dual-end-pumped laser. Output powers of 8.1 W and 7.2 W were realized with 3% and 5% OC transmission, respectively. The slope efficiency amounted to 21% in both cases (Table 1). In comparison to the single-end-pumped experiments with the same lens group, this amounts to an increase of the output power of .
By employing the more efficient coupling lens group 35:200:200, the output power and the slope efficiency were further improved. As shown in Fig. 3(b), this configuration enabled, for the first time, one to reach more than 10 W of output power. A laser output power of 10.1 W was realized under an absorbed pump power of 43.6 W using 3% OC transmission, amounting to 23% optical-to-optical efficiency.
While the dual-end-pumped configuration is advantageous for reducing the lasing threshold and for increasing the output power, it exhibits a lower slope efficiency. To identify the reasons, we separately characterized the forward and backward single-end-pumped resonators. To this end, we investigated the laser using only the p-pol. forward or only the s-pol. backward pump beam. The corresponding laser characteristics are listed in Table 1. The slope efficiencies of the forward pumped lasers were close to those of the single-end-pumped lasers and mostly higher than that of the related dual-end-pumped lasers. In contrast, the slope efficiencies of the backward pumped lasers are lower than those of the single-end-pumped and dual-end-pumped lasers. We believe that the reduced slope efficiencies of the dual-end-pumped lasers were caused by an imperfect spatial matching between the backward pump beam and the laser mode area. Consequently, a higher efficiency and correspondingly higher output power should be feasible by further improving the coupling conditions of the backward pump beam.
While we obtained an output power of 10.1 W in the water-cooled dual-end-pumped resonator, further scaling by increasing the pump power was not successful, which we attributed to thermal effects.
3.2. Laser performance of TEC-cooled lasers
To further explore the power scaling potential of our laser and improve the temperature control, we exchanged the water-cooling of the gain crystal by a thermoelectric cooler (TEC). In this setup, the gain crystal was tightly embedded in a passive copper heat sink glued to the cold side of a TEC, while the hot side of the TEC was glued to a water-cooled copper heat sink. This configuration enabled control of the cooling temperature with a precision of 0.1°C, which ensured a more stable temperature of the laser crystal compared with the directly water-cooled experiments.
With this cooling setup, we repeated the single- and dual-end-pumped laser experiments with the 35:200 and 35:200:200 lens groups, respectively. As shown in Fig. 4, the optimized cooling led to a remarkable improvement of the laser output power compared to the directly water-cooled experiments shown in Fig. 3. In the single-end-pumped scheme, an output power of 10.1 W was achieved under an absorbed pump power of 41.9 W at a slope efficiency of 27% and an optical-to-optical efficiency of 24%. This amounted to a power improvement of more than 25%.
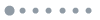
Figure 4.Laser characteristics of the TEC-cooled (a) single- and (b) dual-end-pumped setup resonator with different OC transmissions.
In the dual-end-pumped scheme, the resonator delivered an even 14.1 W of mid-infrared laser output [Fig. 4(b)]. This is the highest output power of any -based, room-temperature, 3-µm CW crystalline laser. The laser exhibited a slope efficiency of 26% at an optical-to-optical efficiency of 24% with respect to the absorbed pump power.
3.3. Further characteristics of the CW lasers
In particular, because -based 3-µm lasers are proned to self-termination, the power stability is a fundamental parameter. Thus, we measured the long-term output power fluctuations at different output power levels. First, we measured the stability of the water-cooled dual-end-pumped resonator at output power levels between 2 W and 8 W using 3% of OC transmission. The stability at the highest power level is shown by the blue curve in Fig. 5(a). As indicated in Fig. 5(b), we found that the instability (PV) decreased from at 2 W to at 8.2 W of output power. As seen in Fig. 6, for lower output powers, dual-wavelength lasing around is typically observed, which may lead to mode competition contributing to the output power instabilities. However, we attributed the main, somewhat regular, pattern in the power characteristics to the heating/cooling cycle of the chiller used for the water cooling of the laser crystal, which motivated further investigations using TEC cooling.
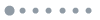
Figure 5.(a) Power instability (PV) of the water-cooled laser at 8 W and the TEC-cooled laser at 12 W. (b) Variation of the power instability (PV) versus the output power of the water- and TEC-cooled lasers.
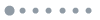
Figure 6.Laser spectra of the TEC-cooled dual-end-pumped resonator at 3% OC transmission at different output power levels.
The long-term output power fluctuations under TEC cooling at 12 W of output power and are shown by the red curve in Fig. 5(a). The progress of the instability versus the output power is shown in Fig. 5(b). The significantly higher temperature stability of the TEC-cooled laser crystals avoids the abrupt and large-amplitude power variations seen for the water-cooled experiments, and thus enables more stable laser output. This means that the periodic fluctuations of the output power in the water-cooled laser can be attributed to the thermal effect. At the highest measured power level of 12 W the instability (PV) of the TEC-cooled laser amounted to only . At power levels around 9 W, comparable to the highest power investigated in the water-cooled experiments, the instability was as low as , which corresponds to an improvement by a factor of 3.5. These results clearly show that the temperature stability of the -doped gain media for 3-µm lasers is a crucial factor for achieving stable laser output power.
The laser spectra of the dual-end-pumped laser using 3% OC transmission at different output powers were measured by an OSA207C Fourier transform spectrum analyzer (Thorlabs Inc.). As shown in Fig. 6, the laser emission wavelength of the TEC-cooled dual-end-pumped lasers changes in the range of 0.25 W of output power. For output powers below 0.2 W, single, dual, and multi-wavelength operation in the 2720 nm region is observed. At 0.3 W of output power, the onset of lasing at 2845 nm is observed and lasing around 2720 nm slowly fades out. At power levels above 0.3 W, 2845 nm is the only laser wavelength. Such a behavior was previously observed[12] and results from the reabsorption of the laser at ∼2720 nm with increasing power levels via the increasing population of the lower laser level. Moreover, we found a slight red shift of the center wavelength with increasing output power, yielding a center wavelength of 2846.1 nm for 13.8 W of output. These observations led us to believe that spectral tuning of the laser output wavelength is feasible.
Finally, we determined the beam quality of the dual-end-pumped resonator at 3% OC transmission by the knife-edge method at different power levels. An uncoated lens with a focus length of 100 mm is employed. A razor blade is fixed on a two-axis manual translation stage (resolution 10 µm) to measure the beam waist in both - and -directions. As indicated in Fig. 7, the factors in the sagittal () and meridional planes () of the TEC-cooled dual-end-pumped lasers gradually reduce from (at 2 W) to (at 12 W).
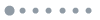
Figure 7.M2 values versus the output power level for 3% OC transmission in the dual-end-pumped water-cooled resonator configuration. Inset: spatial beam characteristics and M2 values at 2 W.
4. Conclusion
In conclusion, we presented our efforts to scale the output power of diode-pumped lasers emitting around 2.85 µm by applying a high-power, narrow-emission pump diode and a dual-end-pumped resonator scheme as well as an improved temperature stability of the gain medium by applying direct TEC-cooling. In a single-end-pumped water-cooled configuration, we obtained an output power of up to 10.1 W under 41.9 W of absorbed pump power, an improvement of more than 70% compared to previous results obtained with [12] and also higher than any other crystalline -based 3-µm laser at room temperature[11]. However, this result was limited by the onset of thermal effects. To homogenize the thermal load and allow for higher pump power levels, we thus applied a dual-end-pumping scheme, and we realized a record high output power of 14.1 W under 59.7 W of pump power at a slope efficiency of 26%. Improving the temperature stability by using a high precision TEC improved the long-term stability of this laser emitting at 2.85 µm to better than at 12 W of output power. Considering slope efficiencies of up to 36% reported in previous work with [12], we anticipate the possibility of output power levels in excess of 20 W with our dual-end-pumped laser scheme by optimizing the pump wavelength, the coupling conditions of the pump source, the output coupler transmission, and the spatial overlap of the pump and the laser beam.