1 Introduction
With the advancement of wireless communication technology, frequency resources in the medium and low frequency bands are becoming increasingly scarce. Currently, the millimeter wave (mmW) band remains relatively underexploited. Due to its wide bandwidth and strong anti-interference properties, mmW has gained favor, and wireless communication technology is gradually shifting towards this frequency band. The spectrum allocation for the fifth-generation (5G) mobile communication network includes the Ka-band (26.5 GHz–40 GHz), which marks the beginning of the mmW band [1]. However, mmW frequencies suffer from significant propagation losses, atmospheric absorption, scattering, and non-line-of-sight losses, limiting communication distances. To compensate for these losses, phased array antenna technology can be employed to meet the demand for rapidly controllable high-gain beams in the mmW frequency band [2–4].
Antennas used in mobile communication terminal equipment generally need to meet conditions such as low profile, lightweight, and fast beam switching [5]. Compared to mechanically scanned phased arrays, electric scanning arrays (ESAs) offer unparalleled flexibility, combined with small size, low cost, light weight, and high reliability [6]. In the implementation of ESAs, loading variable reactance components is a novel and cost-effective approach. Under the premise of a fixed unit structure, when varactor diodes or p-i-n diodes are passed through the edge of the radiating structure, the patch resonance frequency often decreases with the increase of junction capacitance. The reconfigurable feature of utilizing the impedance characteristics of diodes allows for the modulation of the phase characteristics of the antenna. This approach has been applied in Refs. [7–12]. In Ref. [9], a 2-bit phased array antenna utilizing series-parallel feed with p-i-n diodes was proposed for Ku-band one-dimensional beam scanning applications. In Ref. [10], a 3-bit leaky-wave antenna was implemented at 28 GHz by loading extension lines, but it required the use of 8 p-i-n diodes and lacked a complete prototype demonstration with diodes loaded. Reference [11] presented a 4-bit phase shifter operating at 18.7 GHz by cascading four reflective phase shifters, employing 8 p-i-n diodes. Meanwhile, reference [12] introduced a 4-bit reconfigurable antenna element working at 12 GHz, consisting of a 1-bit magneto-electric dipole loaded with 2 p-i-n diodes and a reflective type phase shifter equipped with 4 varactor diodes. Although this array offers nearly continuous phase-matched beam scanning capability, it sacrifices antenna gain due to the high insertion loss caused by diodes and lacks the ability for two-dimensional scanning.
Integrating diodes into antennas is crucial for phase-reconfigurable phased array beam scanning. This method has been widely used in the Ku-band and below, as given in Table 1. However, in the Ka-band, due to the increase in frequency and the corresponding reduction in antenna array size, the manufacturing difficulty increases significantly [13]. Additionally, the loaded diodes and other electronic components may generate parasitic effects and electromagnetic interference with the antenna patches, which can significantly affect the antenna’s radiation performance [14]. Therefore, careful design of the antenna structure with loaded electronic components is required. This article showcases a structurally simple left-hand circularly polarized 2-bit phased array operating at 27 GHz, capable of one-dimensional beam scanning. Based on the proposed element design, a 1×8 array was devised for verification. Experimental results demonstrate that the array can achieve beam scanning within a ±50° range.

Table 1. Comparison between other relevant researches.
Table 1. Comparison between other relevant researches.
Reference | Frequency(GHz) | Element size(λ0) | Array size | Number of diodes per element | N-bit | Scanning range | Complete prototype demonstration | 1 Bold fonts indicate the relevant data for the proposed method. | [7] | 1.5 | 0.5 | 2×4 | 4 | 2 | ±28° | √ | [8] | 3.65 | 0.5 | 1×8 | 4 | 2 | ±49° | √ | [9] | 17.3 | 0.46 | 9×8 | 4 | 2 | ±50° | √ | [10] | 28 | 0.56 | 1×8 | 8 | 3 | ±50° | × | [12] | 12 | 0.52 | 2×16 | 6 | 4 | ±45° | √ | Prop. | 27 | 0.5 | 1×8 | 4 | 2 | ±50° | √1 |
|
2 Design and analysis of 2-bit antenna element
2.1 Ka-band 2-bit antenna element
Fig. 1 illustrates the proposed 2-bit antenna element structure in this article. In addition to meeting the required impedance bandwidth, the antenna element needs to achieve a 1-bit phase resolution at the radiation patch level. Therefore, a simple stacked microstrip antenna topology with probe feeding can satisfy the design requirements of this array.
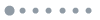
Figure 1.Geometry of the proposed 2-bit element: (a) 3D view, (b) top view, and (c) bottom view. (h1 = 0.508 mm, h2 = 0.1 mm, h3 = 0.203 mm, d = 5.60 mm, l1 = 1.82 mm, l2 = 0.63 mm, l3 = 0.41 mm, w1 = 0.60 mm, w2 = 0.59 mm, l4 = 1.60 mm, l5 = 1.00 mm, l6 = 0.90 mm, w3 = 0.43 mm, w4 = 0.50 mm, w5 = 0.38 mm, w6 = 0.43 mm, and w7 = 0.35 mm)
As shown in Fig. 1 (a), the antenna adopts a 4-layer printed circuit board(PCB)structure, consisting of a radiation patch layer, a direct current (DC) bias line layer, a ground (GND) layer, and a phase shifter layer, from top to bottom. The antenna is composed of two layers of RO4003C dielectric substrates (εr = 3.55, tan σ = 0.0027) laminated together. The thicknesses of the upper and lower dielectric substrates are h1 and h3, respectively. An RO4450F prepreg (εr = 3.2, tan σ = 0.004) with a thickness of h2 is inserted between the two substrates. P-i-n diodes and inductor components are soldered on the surfaces of both the top and bottom layers of the antenna.
In Fig. 1 (b), the radiation patch layer is an angular-cut annular microstrip patch, capable of achieving left-hand circular polarization (LHCP) performance. As the element rotates along the z-axis, the phase of the radiation field will advance or lag according to the direction of rotation. Therefore, by utilizing the rotational symmetry of this design, by reverse parallel connecting two p-i-n diodes (PIN1 and PIN2) on the radiation patch, equivalent 180° phase shifting performance can be achieved.
The 90° reflective phase shifter in microstrip form is shown in Fig. 1 (c). The phase shifter is composed of a circular directional coupler and two parallel p-i-n diodes (PIN3 and PIN4) loaded at its through and coupled ports, along with open-circuit short lines. Changing the on/off state of the p-i-n diodes can alter the impedance at the reflection terminal. By adjusting the length of the open-circuit short lines and the position of the p-i-n diodes, it is possible to achieve two different phases of 0° and 90° by controlling the on/off state of the p-i-n diodes. The phase shifter is cascaded with a center feed probe and radiating patch, forming a “coaxial probe-microstrip line” structure, enabling the antenna to connect with a power divider on the same plane. In the end, a cascaded 2-bit phased array antenna element controlled by four p-i-n diodes and two DC bias lines is realized. As a result, the radiation field phase of the antenna can be divided into four states: 0°, 90°, 180°, and 270°. Table 2 lists the corresponding p-i-n diode states of the antenna in different phase modes, where “0” represents the OFF-state, and “1” represents the ON-state. In the simulation, the states of the p-i-n diode (MACOM, MA4FCP200) are set according to Table 2, where the ON-state and OFF-state are modeled as a series of a resistor (R), an inductor (L), and a capacitor (C) components, as shown in Fig. 2.

Table 2. Antenna status defined by different codes of p-i-n diodes.
Table 2. Antenna status defined by different codes of p-i-n diodes.
Antenna mode | PIN1–PIN4 | Relative phase | State 1 | 1000 | 0° | State 2 | 0111 | 90° | State 3 | 0100 | 180° | State 4 | 1011 | 270° |
|
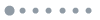
Figure 2.Equivalent circuit of p-i-n diode.
The inductor used in the DC bias circuit is the inductor component with the model number L0201R82AHSTR produced by KYOCERA AVX. This inductor component has a self-resonant frequency of 28 GHz and exhibits excellent isolation performance within the operating frequency range of the antenna proposed in this paper. The bias circuit consists of narrow microstrip lines and inductors, forming a band-stop network in the operating frequency range to isolate RF signals from DC signals.
Fig. 3 presents the antenna surface current distribution at the working frequency of 27 GHz and at the moment of t = 0, with the p-i-n diodes configured according to Table 2, enabling the antenna unit to operate in different mode states. It can be observed from Fig. 3 that under different switching configurations, the surface current of the rectangular patch exhibits different patterns. It is intuitive to see that the electric field phase of the left-hand circularly polarized antenna has differences of 0°, 90°, 180°, and 270°. Therefore, this antenna can operate as both a radiator and a 2-bit phase shifter.
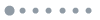
Figure 3.When the antenna unit operates in different mode states, the surface current distributions of the top radiation patch and the bottom phase shifter: (a) state 1, (b) state 2, (c) state 3, and (d) state 4.
2.2 Radiation performance
Based on the analysis above, the radiation performance of the antenna was fully simulated using Ansys HFSS. Fig. 4 (a) shows the simulation results of the port reflection coefficients and the axial ratios (ARs) in four antenna modes. The reflection coefficients of all antenna modes in the range of 26.5 GHz to 27.5 GHz are less than –15 dB. Within the bandwidth range, the ARs of all modes are less than 4 dB. Since sequential rotation was applied to the elements when forming the array, individual elements do not need to have a wide AR bandwidth, which reduces the complexity of element design to some extent. Fig. 4 (b) shows the far-field phase curve of the LHCP electric field in the normal direction. It shows a stable and linear trend of 2-bit phase shift within the operating bandwidth range. The gain patterns of the unit at 27 GHz are shown in Fig. 4 (c) and Fig. 4 (d), and the antenna unit patterns are basically symmetrical in the φ = 0° and φ = 90° planes. A comparison was made to analyze the insertion loss from 4 p-i-n diodes in the antenna by comparing the gain of the complete LHCP antenna, the antenna without the bottom phase shifter, and the antenna without any p-i-n diodes. The complete antenna had a gain of 3.26 dBi to 3.87 dBi. Removing the phase shifter increased the gain to 5.45 dBi, and removing the top p-i-n diodes further raised it to 6.01 dBi. This indicates that the phase shifter causes a loss of 1.58 dB to 2.19 dB, while the top radiating patch with two p-i-n diodes causes a loss of about 0.6 dB.
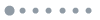
Figure 4.Simulated results under different antenna modes: (a) reflection coefficients and ARs, (b) far-field phases, (c) gain pattern at 27 GHz, φ = 0° plane, and (d) gain pattern at 27 GHz, φ = 90° plane, where CS stands for complete structure, WBPS represents without bottom phase shifter, and WBPSTP represents without bottom phase shifter & top p-i-n diodes.
3 Experimental verification
3.1 1×8 array system
Based on the previously proposed 2-bit phase-reconfigurable antenna structure, a 1×8 array was designed to validate the feasibility of this method. As shown in Fig. 5, the spacing between array elements is 5.6 mm (0.5λ0), where λ0 is the free-space wavelength at 27 GHz. The overall array outline size is 70 mm×33 mm, with a thickness of 0.81 mm. Due to the use of a microstrip structure for the bottom phase shifter, in order to improve the front-to-back ratio, a 1 mm thick metal reflector plate was installed 2 mm below the dielectric substrate to reduce the back lobe level. A ring of metal GND vias was placed around the 1×8 array to form a short-circuit GND structure to prevent surface waves. The DC control line is connected to the DC control port on the outer side of the dielectric substrate, and through an external DC control circuit, the voltage and current of the corresponding ports can be switched to control the on/off state of the p-i-n switch. The radio frequency (RF) input port of the phase shifter is connected to the output port of the 1∶8 Wilkinson power divider. The power divider is connected to the DC GND of the switch via an inductor.
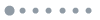
Figure 5.1×8 array exploded view.
To validate the performance of the proposed design, an array sample was manufactured using standard PCB technology. In Fig. 6 (a), the top layer of the array is soldered with a total of 16 p-i-n diodes and 8 inductors. In Fig. 6 (b), the bottom layer of the array is soldered with a total of 16 p-i-ndiodes, 17 inductors, and 7 chip resistors of 100 Ω. The DC bias lines are connected to the 17 pins around the antenna and are connected to the digital control circuit board via DuPont wires. Measurements of the fabricated array were performed in an anechoic chamber; the detailed experiment configuration is illustrated in Fig. 6 (c). A horn antenna operating from 26.5 GHz to 40 GHz was used as the receiving antenna.
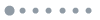
Figure 6.Fabricated array sample: (a) top view, (b) bottom view, and (c) experimental configuration in the anechoic chamber.
3.2 Discussion of measured results
Fig. 7 (a) exhibits the measured |S11| of the array at different scan angles from 26 GHz to 28 GHz. In the antenna working frequency band part (26.5 GHz–27.5 GHz), the measured |S11| is lower than –10 dB. The Gain and directivity of the broadside beams in simulation and measurement are shown in Fig. 7 (b), and they are clearly in good agreement. The simulated directivity is 14.82 dBi and the aperture efficiency is about 58%. The simulated gain is 11.37 dBi at 27 GHz, with a total insertion loss of approximately 4 dB. The insertion loss of the array mainly comes from the following 4 sources:
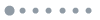
Figure 7.Measured results of the broadside beams at φ = 90° plane: (a) |S11 | and (b) gain and directivity at 0°.
1) Feeding network loss. Through simulation, the loss incurred by the 1∶8 Wilkinson power divider feeding network is approximately 1.3 dB.
2) Phase shifter loss. The 90° reflective phase shifter, equipped with 2 p-i-n diodes, introduces a loss of approximately 1.6 dB to 2.2 dB.
3) Diode loss in the top patch. Compared to a printed electronic circuit (PEC) radiating patch, the radiating patch loaded with 2 p-i-n diodes incurs an additional loss of approximately 0.6 dB.
4) Ohmic loss. The ohmic loss from the radiating patch and feeding probe is approximately 0.2 dB.
As shown in Fig. 7 (b), the measured gain is 10.23 dBi, which is 1.07 dB lower than the simulated gain., respectively. The measured gain is slightly lower than the simulated result, mainly due to two factors: Poor isolation when the p-i-n diodes are in the OFF-state, and additional losses introduced by the Mini-SMP connector to SMA connector adapter during the actual measurement.
The measured scanning radiation patterns from 0° to 50° are shown in Fig. 8. The results verify that the test prototype has good scanning performance. Due to the symmetry of the array structure, the beam scanning capability at negative angles is equivalent to that at positive angles. When the array scans to 50°, the measured gain is reduced by 3.96 dB compared to 0°, and the sidelobe level (SLL) reaches the highest at –4.29 dB. Within the ±50° scanning range, the axial ratio of the array antenna is less than 4 dB. When the antenna scans to a large angle, due to the edge effect of the 1×8 array, the pattern of the edge unit deteriorates at large angles in terms of axial ratio. However, this deterioration can be effectively suppressed when forming a larger array [15].
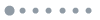
Figure 8.Measured gain patterns of scanning beams at 27 GHz, φ = 90° plane.
Table 3 summarizes the corresponding performance indexes of the array at 27 GHz, including peak gains, SLLs, actual scanning angles, and ARs. Due to the influence of quantized phase errors, there is a slight pointing error in the scanning beam, and as the scanning angle increases, relatively high peak SLLs will appear, leading to increased gain fluctuations.

Table 3. Performance indexes of measured beams.
Table 3. Performance indexes of measured beams.
Scan angle | Gain (dBi) | SLL (dB) | AR (dB) | 0° | 10.23 | –11.39 | 2.05 | 10° | 9.08 | –8.54 | 1.27 | 20° | 8.86 | –6.53 | 2.07 | 30° | 8.85 | –5.95 | 2.80 | 40° | 7.65 | –5.30 | 0.39 | 50° | 6.27 | –4.29 | 3.73 |
|
4 Conclusions
This paper proposes an LHCP 1×8 array antenna operating at 27 GHz with 2-bit phase tuning functionality. The 2-bit phase shifting unit is achieved by cascading a reflective phase shifter with a 90° phase shift function, along with a 1-bit annular radiating patch. Using this 2-bit phase shifting unit, a 1×8 array is constructed by employing parallel forced feeding through a Wilkinson power distribution network. A prototype of the 1×8 array has been successfully produced, which operates in the frequency range of 26.5 GHz to 27.5 GHz and achieves a peak gain of 10.23 dBi at the center frequency (27 GHz). The prototype demonstrates a flexible beam scanning capability of ±50° in the φ = 90° plane and has the potential to be expanded into a two-dimensional planar array.
Disclosures
The authors declare no conflicts of interest.