1. INTRODUCTION
Plasmons are fundamental to the nanoscale manipulation of electromagnetic fields, a key aspect in advancing photonic device [1–3]. Their role is crucial in sensing [4], spectroscopy [5], and nonlinear optics applications [6,7]. There are various methods to leverage plasmonic effects, such as employing metal nanostructures, surface plasmon polaritons, graphene plasmons, and hybrid systems [8–13]. Among them, graphene is notable for its surface plasmon resonance, high conductivity, two-dimensional structure, minimal reflectivity, and option to manipulate the excitation and emission of thermal photons [14–16]. These properties enable significant absorption of plasmonic effects in graphene. However, there are some inherent disadvantages including limited tunability, short propagation distances, and difficulties in coupling with external light sources in graphene plasmons (GPs) [17].
To optimize GPs properties, methods such as doping, introducing defects, structural modifications, and integrating plasmonic nanostructures have been employed [18,19]. These approaches aim to enhance light interaction and absorption across various wavelengths. Metallic gratings stand out as a very promising method to activate GPs, which are efficient in coupling incident light to plasmons and augmenting light–matter interactions [8,20–23]. In addition, metal grating nanostructures offer distinct advantages, categorized by their support for localized surface plasmons (LSPs) and propagating surface plasmons polaritons (SPPs) [2,10,24,25]. LSPs are confined to smaller nanostructures, while SPPs propagate over longer distances along metallic surfaces. Plasmonic metal nanostructures for SPPs offer advantages in field confinement, sensitive sensing, energy transfer, and distance communication. However, there are also some drawbacks of metallic grating structures, such as high energy dissipation, constrained spectral range, polarization sensitivity, challenges in fabrication and material compatibility, and especially the Joule heating leading to significant thermal degradation and reduced device longevity [26–28].
The development of (GSBT), a modification of with Bi, has attracted attention for its phase-changing capabilities, transitioning between amorphous and crystalline states under thermal, electrical, or optical stimuli [29–31]. In addition, graphene-based devices have demonstrated a wide range of applications, such as -switched and mode-locked lasers with high modulation depth and stable pulse generation, but often require precise control over material properties or external configurations to achieve optimal performance [32–34]. The essential absorption properties of GSBT, high thermal stability, and compatibility with graphene render it a very promising candidate for efficient light absorption and heat dissipation in graphene-based devices [35,36]. The van der Waals (vdW) heterostructure of GSBT-graphene (GSBT-Gr) shows particular sensitivity to crystalline and periodic structures. Annealing transforms GSBT into a crystalline state, enhancing light absorption and enabling crystalline GSBT (cGSBT) nanostructures to simulate plasmonic effects akin to metallic nanostructures [37]. This process improves optical nonlinearity and relieves some limitations associated with metallic gratings.
Sign up for Photonics Research TOC Get the latest issue of Advanced Photonics delivered right to you!Sign up now
In this paper, a novel combination of GSBT with Gr thin film vdW heterostructure on gold (Au) film has been proposed and fabricated to activate GPs, LSPs, and SPPs, simultaneously, in which the unique light absorption and nonlinear optical properties of GSBT have been utilized to enhance light–matter interactions. The two-dimensional cGSBT grating-graphene (2D cGSBT-Gr) vdW metasurface was prepared by the laser direct writing (LDW) technique, which can well control the fabrication process of a subwavelength grating structure and can also make the original amorphous GSBT (aGSBT) into metallic cGSBT. The GSBT-Gr vdW heterostructure exhibits a significantly increased optical absorption ability. Integrating it into a fiber laser system enables high-performance mode-locked pulsed laser output. This approach has been demonstrated effectively to mitigate common issues of metallic gratings, such as excessive heat production and energy loss, showing the potential of the cGSBT-Gr vdW combination in various applications of the design and fabrication of plasmonic and photonic materials and devices.
2. METHODS
A. GSBT Micro-Nanostructures with the LDW Fabrication Techniques
To achieve precise excitation of micro-nano grating structures and maximize surface plasmon resonance effects, the LDW fabrication techniques require fine control over material phase transitions and localized enhancement of the optical fields. This involves precisely calibrating LDW system parameters and stringent execution of chemical etching steps. Figure 1(a) illustrates the fabrication sequence of GSBT micro-nanostructures using LDW.
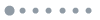
Figure 1.Fabrication process and resultant metasurfaces of GSBT micro-nano gratings. (a) The schematic diagram of the fabrication process of GSBT gratings. Inset: the SEM images of (b) 1D-cGSBT grating and (c) 2D-cGSBT grating.
GSBT, known for its phase-change properties, is an advanced photoresist that undergoes laser-induced crystallization from an amorphous state, which is critical for enhancing light-trapping efficiency in metasurfaces. The process begins with substrate preparation, followed by the deposition of an Au layer. A resist layer is then added, over which GSBT is deposited using magnetron sputtering technology. The LDW for the laser exposure technique is crucial for precisely patterning the GSBT film. It involves targeting selected areas with a controlled laser beam, allowing for high-resolution structuring. Subsequently, wet etching in a solution of nitric acid (), hydrogen peroxide (), and water () is employed to etch the amorphous and crystalline regions of GSBT differentially. The careful preparation of a solution, maintaining the formula , is considered highly crucial. In this experiment, the acid solution is utilized for the corrosion of aGSBT, while cGSBT is left intact, capitalizing on the role of GSBT as a negative photoresist. Leveraging the distinct chemical reactivity, it is noted that the aGSBT, characterized by its abundance of unpaired electrons, shows a heightened reactivity toward protons. This characteristic facilitates selective etching, a vital aspect of the process.
Figure 1(b) presents SEM images of 1D cGSBT grating metasurfaces, which clearly demonstrate the metasurface’s uniform periodicity of 1550 nm and a duty cycle of approximately 0.5, where the width of the grating features measures 750 nm. This fine control in the fabrication process, achieved via LDW, highlights the precise periodic structures that are critical for enhanced plasmonic interactions. Additionally, the SEM image of the 2D GSBT grating structure in Fig. 2(c) shows well-defined square patterns, with each side measuring 750 nm. This use of LDW to create highly uniform two-dimensional nanopatterns underpins the critical role of precise microstructure and nanostructure fabrication in plasmon excitation.
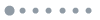
Figure 2.Parameterization of laser power and pulse width in GSBT morphology. (a) A standard checkerboard pattern used for initial experimental setup via LDW. (b) The optical microscope image of the morphological evolution of GSBT under various laser powers and pulse durations.
B. Experiment Parameter for Laser and Etching Time
The experiment has identified the optimal processing parameters for fabricating 1D and 2D grating structures, ensuring the accurate replication of designed 2D nanopatterns for Fig. 2(a). Increasing laser power causes notable morphological changes once the phase transition energy threshold is reached on the aGSBT surface, as depicted in Fig. 2(b). At lower powers with 15 mW, patterns have appeared, with brighter areas indicating the phase transition of aGSBT to cGSBT. This results in a clear contrast with nonirradiated regions. Bright centers and undefined edges occur due to the Gaussian distribution of laser intensity and fine scanning steps, leading to energy overlap at the center. To sharpen the entire pattern, increasing the laser power is necessary so that the edges also undergo the phase transition without the center overheating. While keeping the pulse width constant at 50 ns, it was observed that laser powers above 35 mW led to material degradation. Excessive power not only darkens the pattern but also causes localized melting, ultimately distorting the desired structure. Thus, an optimal laser power of about 25 mW is identified, balancing pattern illumination and edge definition.
Laser pulse width also critically influences pattern definition. Utilizing a 25 mW optimum power value setting, we explored various pulse widths (20 ns, 50 ns, and 100 ns). Clear boundaries are maintained across these settings, with the 50 ns pulse width providing the brightest surface. The 20 ns width is too short for sufficient energy build-up, leading to poor contrast. In contrast, the 100 ns width accumulates excessive energy, pushing the GSBT’s surface temperature toward melting, evident from the darkening pattern. Therefore, a 50 ns pulse width is optimal for subsequent experiments, ensuring sufficient energy exposure without overheating.
Similarly, controlling the etching time is critical. In this study, we investigated the optimal development time necessary for etching plasmonic structures of GSBT using SEM imaging. The etching covers a range from 75 to 110 s, as shown in Fig. 3. Initial findings, depicted in Fig. 3(a) at 75 s, show minimal alterations in the aGSBT material, indicating that insufficient development time prevents etching of this material. This underscores the need for adequate etch time to achieve cGSBT grating, essential for forming functional plasmonic structures. As the development time increases, Fig. 3(b) at 80 s and Fig. 3(c) at 83 s continue to display minimal change, suggesting that these intervals are still below the threshold needed for effective etching of aGSBT. A pivotal observation is made at 85 s, represented in Fig. 3(d), where the optimal etching of cGSBT grating structures is achieved. This etching time is the critical balance between sufficient development time to etch aGSBT while preserving the integrity of cGSBT fully, which is crucial for maintaining the functionality of the plasmonic structures.
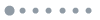
Figure 3.SEM analysis of etching progression over time. (a)–(c) At 75 s, 80 s, and 83 s, the early stage of etching shows the aGSBT material largely unetched, with no significant structural features evident. (d) By 85 s, the cGSBT grating structures reach optimal definition, showcasing effective material transformation. (e), (f) At 87 s and 90 s, the onset of over-etching becomes apparent, leading to the beginning loss of structural integrity.
However, extending the development time beyond this optimal point leads to over-etching. This is evident in Fig. 3(e) at 87 s and Fig. 3(f) at 90 s, where we begin to observe the degradation of cGSBT structures, indicating that excessive development time can compromise the grating’s structural integrity. The time of this etching process is over 90 s, which reveals extensive material removal and a substantial loss of grating definition. These observations highlight the critical need for precise control of development time in the etching process. Properly calibrated, the etching time ensures the complete etching of aGSBT without compromising the structural and functional integrity of cGSBT. This balance is essential for optimizing the performance of plasmonic structures in practical applications, illustrating the nuanced interplay between process time and material response in manufacturing.
3. RESULTS AND DISCUSSION
A. Four Different Devices Based on Graphene
In the past decades, great efforts have been made to boost the GPs. Among them, the GPs coupling of graphene-nanostructures such as graphene/metal ribbon [20,22,38] and graphene/metal hybrid films [8,10] stands out as a very promising method to enhance the light–matter interaction; however, tailoring the plasmonic characteristics of graphene-based hybrid nanostructures is still challenging.
In this work, the pure graphene film and three kinds of graphene-nanostructures have been prepared as a saturable absorption medium to generate short pulse laser operation. The bare graphene, metallic grating-graphene (Au grating-Gr), dielectric film grating-graphene ( grating-Gr), and one-dimensional cGSBT grating-graphene (1D cGSBT-Gr) metamaterials were fabricated by transferring graphene onto the respective substrates using a wet transfer method, with detailed fabrication procedures shown in Fig. 11 (Appendix A). As seen in Fig. 4, when the incident continuous-wave light [in the time domain shown in Fig. 4(a) and frequency domain shown in Fig. 4(b)] passing through the four different samples of bare graphene [Fig. 4(c)], Au grating-graphene [Fig. 4(d)], dielectric film grating-graphene [Fig. 4(e)], and 1D cGSBT-Gr metamaterial [Fig. 4(f)], the resultant frequency and time domain variations are depicted in Figs. 4(g)–4(j) and Figs. 4(k)–4(n), respectively. Under identical working conditions, the vdW heterostructure of the 1D cGSBT-Gr clearly demonstrates the generation of a mode-locked signal, as illustrated in Figs. 4(j) and 4(n), which display the pulse train and spectrum of the mode-locked laser, respectively. Only -switched pulse output was obtained when the same continuous laser passed through the other three samples. These obviously distinct experimental results suggest that the vdW of the cGSBT grating nanostructure with GPs can effectively enhance light–matter interactions and increase the optical nonlinearity and absorption characteristics.
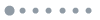
Figure 4.Comparative analysis of graphene-based plasmonic nanostructures and their effects on laser output. The incident continuous-wave light for (a) the time and (b) frequency domains. The light interacts with various graphene configurations: (c) bare graphene, (d) Au grating-Gr, (e) grating-Gr, and (f) 1D cGSBT-Gr metamaterial. The pulse outputs from these structures are captured in (g)–(j) for the time and in (k)–(n) for the frequency domains, respectively. Among them, (g), (k) correspond to bare graphene, (h), (l) to metallic grating-graphene, (i), (m) to dielectric film grating- graphene, and (j), (n) to 1D cGSBT-Gr metamaterial. The vdW heterostructure of 1D cGSBT-Gr metamaterial’s mode-locking capability is evident from its distinctive pulse and spectral features, showcasing the unique plasmonic modulation achieved.
B. Characterization and Simulation of the GSBT Metasurface Structure
To further explore the plasmonic properties of GSBT nanograting structures, an investigation was carried out on four different types of samples: aGSBT, graphene-integrated aGSBT (aGSBT-Gr), cGSBT, and graphene-integrated cGSBT (cGSBT-Gr). Each sample was fabricated on a GaAs semiconductor substrate. A 400 nm Au layer was first deposited on the GaAs substrate by using electron beam evaporation. This step was followed by the deposition of 40 nm, 60 nm, and 80 nm GSBT thin film via radio frequency magnetron sputtering, conducted at radio frequency magnetron sputtering under the condition of 0.1 Pa work pressure, 50 W power, and 25 sccm Ar flow. In this work, the 60 nm GSBT exhibits the best optical absorption capability, so all the following works are based on the sample with 60 nm GSBT film. Then graphene was affixed to the surface by a wet transfer method, and these samples underwent an annealing process in a tubular furnace. The study began with an analysis of cGSBT to investigate the effects of the cGSBT-Gr vdW heterostructure plasmons.
Figure 5(a) displays the XRD patterns of both as-deposited and annealed GSBT films. The as-deposited film displayed an amorphous structure, via subsequent post-annealing at 150°C, and transitioned into a crystalline state as indicated by the emergence of the (200) diffraction peak. The Raman spectra depicted in Fig. 5(b) for aGSBT showcase two broad peaks that are ascribed to the vibrational modes of the Sb-Te and Ge-Te bonds. In contrast, three typical Raman peaks of cGSBT centered at , , and were found in the spectrum, corresponding to the tetrahedron vibrational mode , the vibration of Te-Te bond, and the vibrational mode of crystalline , respectively, which aligned with a different vibrational mode inherent to the crystalline structure [39]. Further complementing this analysis, Fig. 5(c) exhibits additional Raman peaks at and , signaling the presence of high-quality graphene. These peaks, representative of the G-band and 2D-band respectively, affirm the excellent preparation of graphene, with the sharpness and intensity of these bands indicating a structure with minimal defects and high crystalline integrity.
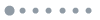
Figure 5.Characterization of GSBT structures and their graphene composites. (a) XRD patterns of as-deposited GSBT and cGSBT. (b) Raman spectra of aGSBT and cGSBT. (c) Raman spectra of aGSBT-Gr and cGSBT-Gr. (d) Absorption spectra of aGSBT, aGSBT-Gr, cGSBT, and cGSBT-Gr. AFM images of (e) aGSBT, (f) cGSBT, (g) aGSBT-Gr, and (h) cGSBT-Gr.
Figure 5(d) illustrates the absorption spectra, revealing a substantial increase in the light absorption capacity of GSBT films upon integration with graphene. Specifically, the absorption rates of aGSBT and cGSBT are enhanced from 16.58% to 40.21% for aGSBT-Gr, and from 39.67% to 68.16% for cGSBT-Gr, respectively. This enhancement underscores the pivotal role of graphene in augmenting the optical properties of GSBT films. AFM images from Figs. 5(e)–5(h) highlight the morphological differences between the films. In comparison to aGSBT [Fig. 5(e)], the cGSBT [Fig. 5(f)] shows a rougher texture due to the coalescence of grains during annealing. At the same time, the addition of graphene contributes to a smoother surface, as observed in Figs. 5(g) and 5(h).
Given the demonstrated capability of graphene to enhance the optical absorption of GSBT, further investigations into the excitation of plasmons within graphene by GSBT gratings were conducted. Figure 6 presents electric field distributions across varied metasurfaces from finite-difference time-domain (FDTD) simulations. Figure 6(a) showcases the simulated electric field distribution of a 1D cGSBT grating, with the corresponding design schematically illustrated in Fig. 6(e) displaying uniform field lines indicative of a standard grating effect. Figure 6(b) shows the field distribution for the 1D cGSBT-Gr, as represented structurally in Fig. 6(f). Compared to the bare cGSBT grating, the slight increment in field intensity for 1D cGSBT-Gr can be attributed to the intrinsic optical absorption properties of graphene, which improve the electric field strength. This interaction is a critical component in the enhancement of GP phenomena.
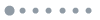
Figure 6.Simulated electric field distributions of cGSBT and graphene-integrated grating metasurfaces. (a) displays the field distribution in 1D cGSBT grating. (b) shows the enhanced field in a 1D cGSBT-Gr, demonstrating the effect of graphene integration. (c) depicts the complex field interactions in a 2D cGSBT-Gr. (d) zooms in on the cross-sectional electric field in the 2D cGSBT-Gr, highlighting the augmented plasmonic interaction. Accompanying schematics for (e) the 1D GSBT grating, (f) the 1D cGSBT-Gr, and (g) the 2D cGSBT-Gr and (h) detail of the cross-sectional view of the 2D cGSBT-Gr. Note: the illustrations of 1D/2D pattern and graphene are not shown to scale.
Figure 6(c) elucidates the electric field distribution for the 2D cGSBT-Gr vdW heterostructure, as graphically represented in Fig. 6(g), which shows field intensity more than 23 times that of the 1D cGSBT-Gr vdW heterostructure. This significant enhancement underscores the efficacy of integrating a nanoscale two-dimensional grating structure with graphene to fortify the effects of GPs and exhibit strong localized surface plasmon resonance (LSPR). An expanded simulation of the cross-section for the 2D cGSBT-Gr vdW heterostructure, represented schematically in Fig. 6(d), reveals strong electric field strength at the edges, indicating that the nanoscale for 2D cGSBT can augment the LSPs within the 2D cGSBT-Gr vdW heterostructure. The width () and height () of the 2D grating are crucial dimensions that contribute to this field strength, as shown in Fig. 6(h), pivotal for advancing plasmonic applications in optoelectronic devices.
C. Working Mechanism of the Metasurface Structure
Figure 7 demonstrates the dynamic evolution of the GSBT structures, which are pivotal in enabling strong plasmon coupling. In Fig. 7(a), the disordered phase of the aGSBT lacks the ordered atomic arrangement necessary for LSPs, which is crucial at the nanoscale for plasmonic activity. The transition in Fig. 7(b) reveals the metamorphosis of GSBT under LDW [40,41], making it a 1D cGSBT grating aligned with graphene at an Au film. This configuration, conducive for GPs and SPPs, marks a significant shift, enhancing plasmon interactions in graphene and facilitating the propagation of plasmonic waves along interfaces. The detailed information on the lattice structures of the GSBT samples before and after annealing process is shown in Fig. 16 (Appendix A). Figure 7(c) shows the LDW technique extends to the and axes, crafting a 2D cGSBT-Gr metasurface for GPs. This patterning strengthens the crystalline material precision, essential for the strong coupling between LSPs and SPPs.
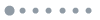
Figure 7.The diagram illustrates the mechanism of a cGSBT-Gr hybrid metasurface, highlighting its structural evolution and plasmonic functionalities. (a) showcases aGSBT with Sb (Bi) atoms forming the apexes of triangular pyramids by three Sb-Te bonds and Ge atoms centered in tetrahedrons by four Ge-Te bonds. (b) displays 1D cGSBT-Gr where Sb (Bi) and Ge atoms are octahedrally coordinated by six Sb-Te and Ge-Te bonds, respectively. (c) illustrates the 2D cGSBT-Gr metasurface fabricated via LDW along both and axes, retaining the atomic coordination of 1D cGSBT-Gr and introducing LSPs effects. (d) depicts the 2D cGSBT-Gr metasurface acting as an SA, where plasmonic interactions transform continuous light into pulsed light, incorporating SPPs effects. The magnified section of (d) highlights the GPs and LSPs. Note: the illustrations of 1D/2D pattern and graphene are not shown to scale.
Figure 7(d) reflects the dynamic interplay and collective coupling among various plasmonic phenomena, GPs, LSPs, and SPPs on a 2D cGSBT-Gr metasurface. This intricate interaction allows the metasurface to act as a saturable absorber (SA), transforming continuous light into pulsed light. The detailed view in Fig. 7(d) illustrates the structure and function of a GP and acoustic GP (AGP) resonator, where the AGP refers to a plasmonic mode with a linear dispersion that is distinct from conventional graphene plasmons and results from anti-phase charge oscillations between graphene and the adjacent metallic substrate, enabling ultrastrong field confinement and enhanced light–matter interactions [22,42,43]. This system includes a cGSBT array positioned under a continuous graphene layer, with a nanogap separating each cGSBT element. The width () and the spacing () contribute to the periodicity () of the array. The unit cell, differentiated by regions with and without underlying cGSBT, supports different plasmonic modes: AGPs in the cGSBT-coupled region and GPs in the cGSBT-free region. The resonance condition of these GPs is described by the equation [22,44] where and denote the momentum of the AGPs and GPs, respectively, and is an integer that represents the order of the resonance. The phases and are nearly zero, indicating a negligible phase shift in the system.
The design of the 2D cGSBT with micrometer-scale features, specifically 750 nm side lengths ( and ), aims to tune the energy of the LSPRs. This tuning is essential for matching the momentum between the incident light and the SPP modes on metal surfaces. The SPP wave vector is satisfied by [23]
In the SPP plasmonic system, specifies the angle at which light impinges on the structure, and are the unit reciprocal lattice vectors of a periodic structure, and and are the periods. The integers and help determine the specific direction of the propagation of the SPP waves. This wave is a fundamental aspect of the interaction between the light wave and the periodic structure, affecting how light is manipulated at the nanoscale.
D. Optical Nonlinear Characterization
To explore plasmonic responses, we test the nonlinear characteristics of various GSBT samples, employing a bespoke nonlinear optical system as depicted in Fig. 8(a). The setup consists of a custom-built femtosecond laser (fs laser) operating at 1550 nm with a fundamental repetition rate of 55 MHz. The system is pumped by a 976 nm laser diode (LD), which is connected to the femtosecond laser using a wavelength-division multiplexer (WDM). A 0.78 m erbium-doped fiber (EDF) serves as the gain medium. The laser output is split using a 50:50 optical coupler (OC). One beam is directed onto various GSBT samples through a circulator (CIR), while the other beam is used for power monitoring () as a reference. The power of the beam interacting with the samples is monitored using a power meter (). By continuously adjusting the power of the 976 nm pump source, the nonlinear reflectivity of the samples was measured at different incident power intensities. This system tested the nonlinear properties of aGSBT (S1), cGSBT (S2), aGSBT-Gr (S3), and cGSBT-Gr (S4) samples, as shown in Figs. 8(b)–8(e). The modulation depths in these samples display a consistent upward trend, and the values recorded were 3.1%, 4.8%, 5.2%, and 6.7%, respectively. This increase in modulation depth correlates with the gradual enhancement of absorption properties, as depicted in Fig. 5(d). This notable correlation sheds light on the impact of material composition on plasmonic responses. Furthermore, it underscores the significant role of GPs integration in amplifying these effects. Further increase of modulation depths of 7.5% and 7.8% was found for 1D cGSBT-Gr (S5) and 2D cGSBT-Gr (S6) samples, as shown in Figs. 8(f) and 8(g).
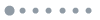
Figure 8.The nonlinear optical characteristics of different GSBT samples. (a) presents the setup of the nonlinear optical system used for testing. The nonlinear optical characteristic of (b) aGSBT, (c) cGSBT, (d) aGSBT-Gr, (e) cGSBT-Gr, (f) 1D cGSBT-Gr, and (g) 2D cGSBT-Gr.
Figure 9 shows the calculated modulation depth and absorption across samples S1 through S6. The blue line with circles represents modulation depth, while the red line with stars indicates absorption. Both metrics show increasing trends across these samples. This pronounced increase in the modulation depth for the grating-integrated samples indicates the heightened plasmonic interaction facilitated by the structured graphene inclusion. The 2D grating, in particular, exhibits a remarkable ability to intensify plasmonic resonance, pointing to the intricate geometry of the grating playing a substantial role in the plasmonic enhancement.
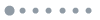
Figure 9.Modulation depth and absorption trends for a series of samples (S1–S6): aGSBT, cGSBT, aGSBT-Gr, cGSBT-Gr, 1D cGSBT-Gr, and 2D cGSBT-Gr.
E. Laser Output Characteristics
The remarkable nonlinear absorption properties of the 2D cGSBT-Gr metasurfaces were integrated within the cavity of a fiber laser system to facilitate the generation of ultrashort pulses. The schematic representation of this integration is outlined in Fig. 10(a), where each component’s role is pivotal in shaping the pulse characteristics. Figure 10(b) shows a direct proportionality between the mode-locked laser’s output power and the pumping power, with a slope efficiency of 0.45%. This efficiency is a testament to the 2D cGSBT-Gr metasurfaces’ capacity to convert pump energy effectively into laser output. The pulse train showcased in Fig. 10(c), with intervals of 286 ns within a 2000 ns span, confirms the attainment of a stable mode-locked regime. The central wavelength of the mode-locked laser, located at 1560 nm, is shown in Fig. 10(d). The stability of the pulse train is further corroborated by the radio-frequency spectrum analysis, with Fig. 10(e) displaying a single peak at a repetition rate of 3.5 MHz, accompanied by a robust signal-to-noise ratio of 66 dB. The autocorrelation trace in Fig. 10(f) and the resultant pulse duration of 1.17 ps, obtained through Gaussian fitting, exemplify the precision and ultrafast nature of the pulse formation.
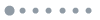
Figure 10.Schematic and results of the mode-locked fiber laser system. (a) Configuration of the mode-locked fiber laser integrating GSBT-Gr as the SA. (b) Demonstrated output power as a function of the pump power. (c) Temporal sequence of emitted pulses in a train. (d) Optical spectrum of the mode-locked laser. (e) Radiofrequency (RF) spectrum highlighting pulse repetition stability. Inset: broadband RF spectrum exhibiting overall system stability. (f) Autocorrelation trace detailing the temporal pulse width. (g) Long-term optical spectrum stability, showcasing the robustness of the laser operation.
Notably, the spectral stability over time, depicted in Fig. 10(g), accentuates the reliability and potential of the 2D cGSBT-Gr metasurfaces for consistent operation in application environments. A 980 nm LD provides the pump power for a 1.1 m EDF, which serves as the gain medium. A 980/1550 nm WDM facilitates the connection between the LD and EDF. The 980 nm LD can supply adjustable pump power ranging from 0 to 800 mW. To maintain optimal polarization within the mode-locked cavity, a polarization-independent isolator (PI-ISO) is positioned between the EDF and the polarization controller (PC). This configuration helps control the polarization state of the light. An OC with 10/90 then splits the laser output, directing 10% of the power for pulse output while the remaining 90% is routed to the CIR and back into the 1550 nm port of the WDM. The 2D cGSBT-Gr gratings, depicted in the SEM image of Fig. 1(c), showcase a bidirectional periodicity that enriches the incident light of the graphene and gold film with 2D cGSBT-Gr gratings. This bidirectional structuring enhances graphene plasmons and guided-mode resonances, allowing for amplified light–matter interaction within the grating structure. The measured 2D cGSBT-Gr of modulation depth with 7.8% signifies the integration’s efficacy and hints at the latent plasmonic synergies at play, which could be leveraged to refine the nonlinearity further and broaden the horizons of GSBT materials for advanced photonic application.
4. CONCLUSION
In summary, plasmons are the quasiparticles resulting from coupling electromagnetic waves with electrons, which play a pivotal role in enhancing the light–matter interaction at the nanoscale. The vdW heterostructure presented in this work ingeniously integrates a 2D cGSBT grating layer with Gr thin film to activate GPs, LSPs, and SPPs simultaneously. This combination results in a remarkable increase of modulation depth of 7.8% that obviously exceeds the 3.1% modulation depth of bare cGSBT. This notable enhancement facilitates the creation of a stabilized mode-locked laser with a pulse width of 1.17 ps and a signal-to-noise ratio of 66 dB. The synergistic combination of GPs, LSPs, and SPPs in the vdW heterostructure composed of phase-changing materials and very thin 2D materials has demonstrated the potential to be utilized to boost the device performance in various research areas of nanotechnology and photonics.