0 Introduction
Mid-infrared waveband contains the characteristic spectral lines of many important molecules and covers important atmospheric transmission windows. Therefore, the mid-infrared laser source has become a research hotspot and has excellent development prospect and potential applications in the fields such as medical diagnosis, biological engineering, military weapons and atmospheric sensing[1-3]. Generally, mid-infrared waveband lasing is generated mainly in several ways. The first is to generate directly by antimonide band-forbidden semiconductor materials. The second is by nonlinear frequency conversion through crystals, such as optical parametric process which can transfer short-wave energy to mid-infrared wave[4]. The third is to generate by pumping rare-earth ions which are doped in low phonon energy glass fiber, crystal or II-VI semiconductor materials[5].
Compared with traditional solid and gas lasers, fiber laser is a good choice for mid-infrared source due to its small size, simple structure, good beam quality, good heat dissipation and so on. At present, mid-infrared fiber lasers at 2 μm waveband are becoming mature by doping thulium-[6-8] or holmium-doped ions[9-11], and the main gain fibers around 3.0 μm waveband use erbium-doped, dysprosium-doped[12-14] or holmium-doped ions[15].
Many results have been reported on 2.8 μm erbium-doped fiber laser. The first report about erbium-doped fluoride fiber laser appeared in 1989 when Alain et al[16] generated 2.71 μm laser by pumping single-mode erbium-doped fluoride fiber with 476, 501 and 647 nm light, respectively. With the in-depth study of erbium ion energy level transition structure and absorption spectrum, scientists found that 976 nm laser is the best choice for pumping erbium doped fiber to generate mid-infrared lasing. With the development of semiconductor lasers as pump source and the maturity of fluoride fiber fabrication technology as gain material, the power of 3 μm waveband fiber lasers has been greatly improved since the 21st century. In 2021, Newburgh et al[17] successfully increased the average power of 2.8 μm erbium-doped fluoride fiber laser to 70 W, which is the highest record in this waveband till now.
Although the development of 2.8 μm waveband laser is rapid, the mechanism and characteristics are still being explored. In this work, a simple linear cavity structure based on highly erbium-doped fluoride fiber is used to investigate the characteristics of lasing, including the forward and backward amplified spontaneous emission (ASE), single and multiple wavelength lasing, optimization of output power and slope efficiency. The full-band emission covering all the emitting lines of erbium ions from 0.5 μm to 3.0 μm was observed and analyzed. Wavelength tuning of 70 nm from 2714 nm to 2784 nm was obtained with pump power increasing from 2 W to
4 W. The spectral splitting was also observed. The lasing at 2.8 μm with the maximum output power of 0.7 W and slope efficiency of about 12% were obtained finally, with the fluctuation of output power less than 1% in one hour.
1 Principle and experimental setup
The simplified energy level transition structure of erbium ion is shown as Fig. 1. According to the absorption characteristics of erbium ions, the typical wavelengths of 650, 790 and 980 nm are used as pumping for erbium-doped fluoride fiber. When 650 nm and 790 nm pumping are used, the ions in ground energy level 4I15/2 transit to energy level 4F9/2 and 4I9/2 firstly, then transit to the upper energy level 4I11/2 through a short radiation-free relaxation. While 980 nm pumping can excite the ground state particles to energy level 4I11/2 directly, and has higher quantum conversion efficiency, therefore this pump source is adopted in this work. The radiation from energy level 4I11/2 to 4I13/2 results in 2.8 μm mid-infrared lasing. The main problem in this process is that the lifetime of the upper energy level is shorter than that of the lower. With pump power increasing, the lower energy level could accumulate a large number of particles, resulting in self-termination of the lasing transition[18, 19], which increases the requirements for pumping rate and laser threshold and reduces the lasing efficiency. In order to overcome the bottleneck problem of particle number inversion, three solutions have been proposed. The first is to generate the laser by cascading the excitation of 1.5 μm and 3 μm waveband, because the excited emission of 1.5 μm laser occurs between energy level 4I15/2 and 4I13/2, which can effectively consume the particle number of 4I13/2 energy level and contribute to the formation of particle number inversion[20]. The second is to use erbium-praseodymium co-doped fluoride fiber, where the erbium ions act as activation ions while praseodymium ions act as the passivator, which can consume the number of particles in energy level 4I13/2 by energy transfer[21]. The third, adopted in this work, is to use highly erbium-doped fluoride fiber to enhance the process of energy transfer up-conversion (ETU) between erbium ions due to the highly doped concentration of ions[22]. The ETU process mainly includesETU1 (energy level 4I13/2 , 4I13/2→4I15/2 and 4I9/2) and ETU2 (energy level 4I11/2, 4I11/2→4F7/2 and 4I15/2 ). This method has been proved to be able to overcome the particle number bottleneck of the lower energy level and reform the particle number inversion. Thus, the stable 2.8 μm mid-infrared continuous-wave lasing could be realized, and the efficiency could be improved significantly.
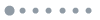
Figure 1.Simplified energy level transition of Er3+
Based on the mechanism aforementioned, we used high-concentration erbium-doped fluoride fiber to build a 2.8 μm mid-infrared fiber laser, as shown in Fig. 2. A 976 nm multi-mode semiconductor laser is output through a silica fiber with a core diameter of 200 μm and numerical aperture of 0.22. A lens (L1, Thorlabs, LA1131-B) with the focal length of 15 mm and optical aperture of 25.4 mm was used for collimation. Then, a CaF2 lens (L2, Thorlabs, LB5766) with a focal length of 50 mm and optical aperture of 25.4 mm was used to focus the pump light into the inner cladding of the fiber. A dichromatic mirror (DM) was inserted between L1 and L2 with an angle of 45° to the direction of pumping. The reflectivity of the dichromatic mirror varying with wavelength is shown in Fig. 3. The transmittivity to the pump light is about 88% and the reflectivity to the 2.8 μm lasing is about 90%. Before coupling into the fiber, the overall transmittance of pump light can reach 82.5%. A Fourier transform spectrometer (ARCspectro Rocket 2.1) was used to measure the output lasing with a maximal resolution of 4 cm-1. The fiber used in the experiment is Er3+: ZBLAN fiber (Le Verre Fluoré, France). The length of gain optical fiber is 4 m, the doped concentration is about 7 mol%, the core diameter is about 0.15 μm, and the numerical aperture is about 0.12. The inner cladding of the fiber is a 240 μm × 260 μm double-truncated circular, which can ensure the fiber to be pumped efficiently. The outer cladding diameter is 290 μm. A gold-plated mirror (M1) with high damage threshold was attached to the other fiber end. The resonator as shown in Fig. 2 is formed by the reflection between M1 and the left fiber end with about 4% Fresnel reflection.
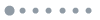
Figure 2.Schematic of mid-infrared erbium-doped fiber laser
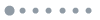
Figure 3.Reflectivity curve of dichromatic mirror
2 Results and discussion
When the pump power reaches 300 mW, the ASE starts to appear. The forward and backward ASE light have different spectral intensity and peak wavelength, which changes with the pump power. Fig. 4(a) shows the spectrum of forward ASE versus the pump power. With the pump power increasing, the spectral intensity increases gradually and the peak wavelength of the spectrum increases from 2.67 μm to 2.75 μm. Fig. 4(b) shows the variation of the backward ASE with pump power. The spectral intensity of the backward ASE increases more quickly than that of the forward ASE and is several-order higher than that of the forward ASE. The possible reason is that the forward ASE only relies on the two fiber ends to form the resonance, which means that M1 was removed in the measurement. In addition, the cause of difference between forward and backward ASE may be related to the longitudinal distribution of pump light power. Compared with forward ASE, the amplification of backward ASE was adapted to the required pump power distribution, resulting in higher transition efficiency, spectral intensity and output power. Fig. 5 shows the variation of peak wavelength of the forward and backward ASE with pump power. The full-width at half maximum (FWHM) of forward ASE is about 9 nm. The peak wavelength of backward ASE is always at 2.714 μm. The FWHM of which ranges from 10 nm to 34 nm.
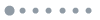
Figure 4.ASE spectrum versus pump power. (a) Forward ASE; (b) Backward ASE
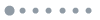
Figure 5.Peak wavelength variation of forward and backward ASE with pump power
When the pump power reaches 1.1 W, stable 2.8 μm continuous-wave lasing begins to appear. After sufficient excitation to produce a stable mid-infrared continuous-wave lasing, the output characteristics are studied. According to the theory of energy level transition, the absorption of erbium ions leads to the change of ion concentration of different energy levels during the energy transfer process, and the difference between transition energy levels determines the emitting wavelength. According to the energy level transition of erbium ion in Fig. 1, from the ground state energy level 4I15/2 to the highest energy level 4H7/2, each level has the possibility to radiate the lasing with corresponding wavelength. Although single-wavelength pumping is used in this work, the phenomenon of full-band lasing emission from 0.5 μm to 3.0 μm still appears. Here the word "full-band" means that the spectrum covers all the emitting lines of erbium ions. Fig. 6 show the full-band output spectrum when the pump power is 3.75 W. Ten peaks appear in the full band, and the emitting wavelengths agree well with the transition between the corresponding energy levels, which are marked in Fig. 6. Most of the lasing lines in Fig. 6 are generated by the transition from some upper energy level to the ground state energy level, except for a few by the transition between two intermediate energy levels. The spectral intensity indicates the emitting strength of the lasing. For the full-band emission, the 2.8 μm lasing is dominant. It is noteworthy that there is no emission generated at the common wavelength of erbium ion in 1.5 μm waveband in the full-band emission spectrum as shown in Fig. 6. This may be due to the high doping concentration of erbium-doped fluoride fibers used in this work. Originally, in the process of ETU1 (energy level 4I13/2 , 4I13/2→4I15/2 and 4I9/2), transitions to higher and lower energy levels occur simultaneously, but in the case of high concentrated erbium ions, the transition to level 4I9/2 and then relaxation to level 4I11/2 dominates, and the transition to the ground state is suppressed, resulting in no 1.5 μm laser emission. This is why erbium-doped fluoride fiber with high doping concentration are chosen in this work.
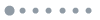
Figure 6.Full band emission spectrum at pump power of 3.75 W. (a) From 0.5 μm to 1.0 μm; (b) From 1.0 μm to 3.0
Fig. 7 shows the evolution of output spectrum with pump power changing from 1 W to 7 W. Fig. 7(a) shows the output spectrum evolution when the pump power increase from 1 W to 2 W. Single-wavelength lasing was generated with the peak wavelength at around 2711 nm and the FWHM of 3 nm. The side-mode suppression ratio increases with the pump power increasing. When the pump power increase from 2 W to 3 W, the wavelength of output lasing starts to shift, but the direction is not monotonic, as shown in Fig. 7(b). The peak wavelength could be tuned in the range of 2714-2774 nm with pump power changing, with the tunable range of 60 nm, as shown in Fig. 8(a). While the FWHM is kept around 3 nm. This tunability by pump power is believed to be caused by the gain competition combined with the selectivity of cavity. When the pump power increase from 3 W to 7 W, the output spectrum undergoes a process from splitting into two peaks to merging into a stable single peak. As shown in Fig. 7(c), at the pump power of 3.25 W, two peaks appear at 2714 nm and 2784 nm, which is caused by the transition competition in band formed by degeneration of energy levels. The peak at 2714 nm has the same wavelength as the pump power at 3 W. With the pump power increasing to 3.5 W, the peak at 2714 nm disappears because it has lower cavity gain in the competition, while the peak at 2784 nm becomes dominant and keeps stable. With the pump power increasing further, the peak at 2784 nm becames stronger and stable. The reason why the peak wavelength varies with the increase of pump power is that the gain fiber has a certain non-uniform broadening effect as well as uniform broadening effect. When the pump power is low, the laser power in the cavity is not high, and the gain of the Er: ZBLAN fiber is not saturated, resulting in the unchanged laser center wavelength. With the pump power increasing, the gain saturation is strengthened and accompanied by certain non-uniform gain broadening, which causes the phenomenon of gain hole burning. The width and depth of burnt holes are different with wavelength, resulting in the laser excitation at other wavelengths. When the pump is increased further, the wavelength of the newly excited lasing will become saturated gradually, and the uniform broadening effect at this wavelength will suppress the original excitation wavelength conversely. When the pump power exceeds 3 W, both uniform and non-uniform gain broadening effect at the peak wavelength of 2784 nm would clamp the gain spectrum in the cavity, resulting in no change of wavelength with the pump power increasing to 7 W. The split spectrum is an intermediate state in the process with pump power increasing. The FWHM of 2784 nm peak is around 2 nm.
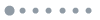
Figure 7.Evolution of output spectrum with pump power. (a) From 1 W to 2 W; (b) From 2 W to 3 W; (c) From 3 W to 7 W
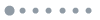
Figure 8.Peak wavelength versus pump power
The variation of peak wavelength with pump power changing from 1 W to 7 W is shown in Fig. 8. The peak wavelength is kept around 2711 nm for the pump power lower than 2 W, while it is kept around 2784 nm for the pump power higher than 4 W. In the range from 2 W to 4 W, the peak wavelength varies in the range from 2711 nm to 2784 nm due to the gain competition in cavity.
The variation of output power with pump power are shown in Fig. 9. According to the fitting results, the slope efficiency of output power is about 12%. As shown in Fig. 10, the stability of output lasing was measured every 5 minutes within one hour, when the pump power was fixed at 6 W. The fluctuation of output power is less than 1% in one hour.
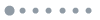
Figure 9.Output power variation with pump power
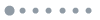
Figure 10.Stability of output power measured in one hour
3 Conclusion
The experimental properties of mid-infrared fiber laser were systematically investigated using highly erbium-doped fluoride fiber. The wavelength tuning of ASE spectrum was measured. The full-band emission covering all the emitting lines of erbium ions from 0.5 μm to 3.0 μm was observed. It is found that the emitting wavelengths agree well with the transition of energy levels. The reason that 2.8 μm lasing is dominant was analyzed. The tunable range of 70 nm was obtained through changing pump power to control the gain competition combined with the selectivity of cavity. Finally, the mid-infrared lasing at 2.8 μm with the maximum output power of 0.7 W and slope efficiency of about 12% was obtained. The fluctuation of average power is less than 1% in one hour. The generated continuous-wave mid-infrared lasing will have applications on laser surgery, gas detection and so on. The results have positive significance for improving the properties of mid-infrared fiber laser.