Hydrogen is the lightest element and exhibits superior mobility under high pressure–temperature (P–T) conditions. Hydrogen, once it has lost its only electron, is electronically equivalent to the proton and can substitute at cation sites in minerals, which in turn enhances their ionic conductivity through the Grotthuss mechanism, or “hydrogen hopping,” in which a proton or proton defect diffuses through the crystal lattice by the formation and concomitant breaking of hydroxyl bonding. Grotthuss-type diffusion is dominant for H-incorporated silicate in the asthenosphere, particularly in regions under relatively high-temperature and low-pressure conditions.1 With increasing depth, H may be liberated from hydroxyl bonding and diffuse freely in the host crystalline lattice, entering an exotic superionic phase.2,3 The concept of a superionic phase is borrowed from the electric battery industry, and the existence of such a phase in ice is widely recognized. The recent discovery of superionic ice–silica in the interiors of giant planets suggests that superionic phases may be common in planetary deep interiors.4 The electrical and seismic features of superionic phases are of great importance, and they have been the subjects of recent studies.2,4 However, what is more challenging is the nature of H in these exotic “semi-fluid” like phases. Will superionicity induce distinct behavior in the distribution of H in major mineral phases? What is the role of proton transportation in the convection of materials? Will it have a large-scale impact?
We take superionic FeOOH as an illustrative example, since this is one of the few superionic phases that have been experimentally confirmed. From a survey of data in the literature, we have found that the pressure and amount of escaped H (nH) are correlated (Fig. 1). Here, we define nH as the fraction of H that has escaped from FeOOH, with nH = 0 for fully hydrous FeOOH, and nH = 1 for complete H depletion. Escape of H is inhibited by increasing pressure, and nH converges to a minimum value of ∼0.2 at the pressure of the core–mantle boundary (Fig. 1), which is consistent with a recent kinetic experiment involving the heating of FeOOH and the prediction of FeOOH0.75 as a stable stoichiometry.5 The regression of P–nH indicates that cross-boundary diffusion is more intense at relatively low pressures.
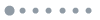
Figure 1.Escaped hydrogen as a function of pressure. Colored open circles are data points from Refs. 2 and 6. Filled circles represent the maximum nH at similar pressures and were selected for a second-order polynomial fit (dashed line, R2 = 0.96). Only data points with pure or dominant pyrite-type phase were picked for the fit. For example, the two outliers at ∼110 GPa were excluded from the fit because their x-ray diffraction patterns revealed the co-existence of contaminated phases.
Cross-boundary diffusion of H is of critical importance and must be taken into consideration in the context of mineral physics for the following reasons. First of all, interdiffusion of heavier elements (e.g., Fe and Mg) in the solid state is extremely slow in the lower mantle: for example, it is only 10−17 m2/s at 26 GPa and 2173 K.7 If such solid–solid diffusion were to dominate mantle convection, then chemical equilibrium by diffusion would be negligible beyond a few meters, even over the entire history of the Earth. In stark contrast, diffusion rates in superionic FeOOH are orders of magnitude higher and thus substantially shorten the time required for chemical equilibrium to be achieved.2 An exotic feature of superionic phases is that the H atoms move freely in the host crystal lattice. In this scenario, the H atom will constitute the unit of mass and energy transportation. Rapid diffusion of H atoms may accelerate the local exchange of energy and convection of materials, making these much faster than in the case of solid–solid diffusion. Second, it is possible to bridge H transportation between superionic phases and nominally anhydrous minerals (NAMs) through cross-boundary diffusion. Although the behavior of H in NAMs should follow the Grotthuss mechanism and will be slower than within a superionic phase, both ionic and superionic diffusion are still considerably faster than solid-state interdiffusion. However, the question arises as to whether superionic behavior will dominate mantle convection, at least in the deep mantle. On the basis of calculated diffusion coefficients in superionic FeOOH (D = 10−9.5–10−7.5 m2/s at relevant P–T conditions), the diffusion length () will only be 13–130 km over the entire history of the Earth. Interconnected FeOOH alone, being a minor phase, will only be one of the factors affecting local material convection and creating small- to medium-scale electrical heterogeneity. It should be noted that in superionic ice, the diffusion coefficient D is one to two orders of magnitude higher than that in FeOOH.8 Given an appropriate host cell and P–T conditions, H diffusion in other hydrous superionic phases, possibly superionic silicates, has the potential to be highly effective in the Earth’s deep interior. If the mass transport of H turns out to be a signature of superionic concentrated regimes, local geochemistry will be better represented by the hydrogen fugacity, since the migration of electrons induced by H will be much more efficient. This novel perspective on the transportation of H will allow exotic stoichiometric phases such as FeO2 and (Mg,Fe)O2 to survive in relatively reduced environments and accumulate as deep oxygen reservoirs.9
The superionic transition breaks H chemical bonds and splits the H2O of hydrous minerals into diffusive H and immobile O, which have been recognized as altering the redox chemistry in the deep lower mantle. Despite the minor presence of FeOOH, given the appropriate P–T conditions, superionic behavior should be common in hydrous minerals. Predicting new mineral phases that exhibit superionic behavior has become an important focus of on-going research. However, it is even more urgent to study the partition of H between the superionic phase and surrounding major lower mantle NAMs. Hydrous minerals can hydrogenate the mantle locally through cross-boundary diffusion, and can even infiltrate to the core if H is siderophile under the conditions at the core–mantle boundary.10 The role of H will have to be revisited by introducing the superionic phase, which may cast new light on large-scale H cycling in the Earth’s deep interior.
Acknowledgment. We thank Yanhao Lin for helpful discussions. Q.H. is supported by a CAEP Research Project (Grant No. CX20210048) and a Tencent Xplorer prize. The work is also partially supported by the National Natural Science Foundation of China (NSFC) Grant No. U1930401.