1. Introduction
Emerging from ultrafast pulse lasers, optical frequency combs (OFCs) have been extensively applied in various fields over the past two decades, including optical atomic clocks[1–4], coherent communication[5,6], spectral analysis[7–9], and lidar[10,11]. It is greatly demanded in both cutting-edge scientific research and engineering technology applications. Historically, OFCs are typically based on mode-locked fiber lasers, which boasted remarkable performance and mature system architecture but were plagued by serious limitations concerning volume, power consumption, cost, and system maintenance[12]. In recent years, whispering gallery mode (WGM) microcavity-based OFCs have sparked a research boom because of their compact size, low structural complexity, and independence of the gain medium. Moreover, microcombs can support a wide range of repetition frequencies from gigahertz to terahertz by flexibly selecting the free spectral range (FSR) determined by the cavity size. Owing to the ultra-high Q factor and the strong field enhancement effect of optical microcavities, microcombs can be acquired with a threshold power as low as sub-milliwatt [13]. This may break the bottleneck of volume and power consumption in traditional OFCs, enabling chip-scale integration and miniaturization of microcombs.
Ultra-high-Q optical microcavities () have garnered increasing interest by virtue of their outstanding nonlinear optical properties[14]. The first observation of optical parametric oscillation (OPO) based on WGMs was reported in 2004[15,16]. Subsequently, discrete comb modes were discovered in a monolithic toroid microcavity when pumped with the continuous-wave laser [17]. Several years later, the spontaneous formation of dissipative solitons in a microresonator was demonstrated, ushering in a new era of portable, low-threshold, and cost-effective soliton microcomb systems[ 18]. More recently, the feasibility of low complexity and budget-friendly production of chip-scale microcomb systems has been revealed. A dual-cavity configuration that combined the laser and microresonator was demonstrated on an integrated hybrid platform, resulting in low-noise soliton-mode-locked combs[19]. Shen et al. presented a turnkey operational regime that can generate solitons immediately upon activation of the pump laser[20]. Xiang et al. first reported on heterogeneously integrated laser soliton microcombs on monolithic Si substrate[21]. Soon after, the synergy of an aluminum gallium arsenide (AlGaAs)-on-insulator (AlGaAsOI) microresonator and silicon photonics (SiPh) integrated components was introduced for optical data transmission and microwave photonics[22]. Concurrently, investigation attention on ultra-low noise photonic signal generation utilizing off-chip microcavities has been pursued and evidenced in succession[23,24]. The latest research has concluded that low-noise photonic microwave generation with ultra-low threshold power can be realized in millimeter-sized devices by drastically improving Q factors and enlarging mode volumes of silica microresonators[25]. Also, based on the highly uniform extinction ratio and absence of mode interactions of microcavities’ transmission spectra, the K-band microwave with ultra-low phase noise can be generated[26]. Although tapered-fiber-coupled discrete microcavities cannot be as integrable and compact as that of the on-chip microresonators, they possess compelling advantages of ultra-high Q factors, mitigated thermal noise, wide repetition frequency range, better fiber compatibility, and lack of high on-off chip loss, which are significant obstacles for on-chip devices utilized in microcombs. However, the stability of the discrete microcavity is still being questioned[27]. In particular, the Q factors may be seriously degraded when microcavity devices’ steadiness and sealability cannot be assured. The resonant property, imputed to its coupling method, is susceptible to ambient vibration, temperature, and humidity fluctuations. These can restrict discrete-microcavity-based OFCs from development and application.
In the realm of microcomb research, the pursuit of practicality presents itself as both an opportunity and a challenge along the path of advancement, particularly within potential implementation domains such as vehicular environments, outdoor exposure settings, and aerospace applications. Consequently, it is an essential step to develop modular packaging technology for discrete microcavities to isolate external disturbances and increase the stability of the coupling structure. The spot-packaged method[28] and full-encapsulated packaging[29,30] use low-refractive-index polymers to actualize device-based packaging of microcavities. Given the high requirements of the dispensing process for the spot-packaging method, as well as Q factor reduction and aging of packaging materials caused by full encapsulation, an indirect packaged microsphere-taper coupling system was proposed[31]. It is worth noting that, in addition to the waveguide coupling scheme, a packaged microresonator coupled with a coupling prism has been realized as a commercial product of the company OEwaves[32]. This coupling method increases system complexity and assembly difficulty, limiting the large-scale application of microcavity technology. Currently, studies on high Q factor packaged microcavity devices have yielded relatively fruitful achievements, yet there are few reports on long-term stability experiments of the Q factors and resonant properties after packaging, which makes our work meaningful.
Sign up for Chinese Optics Letters TOC Get the latest issue of Advanced Photonics delivered right to you!Sign up now
In this paper, we propose a chip-scale ultra-high-Q packaged microcavity device for soliton generation with Q factors up to and good parameter consistency. Additionally, the durability, vibration, and temperature shock experiments of the packaged microcavities are conducted to verify the thermodynamic characteristics and environmental robustness of the proposed packaging method. After that, we demonstrate the generation of lower threshold and mitigated phase noise solitons based on the nonlinear Kerr effect in packaged microcavity devices. This study will significantly accelerate the proliferation of WGM-based practical soliton light source devices.
2. Fabrication and Packaging Technique
Ultra-high-Q microcavities are fabricated through an automated process that involves irradiating lasers onto dehydroxylated high-purity fused silica microrods mounted on a rotating spindle to form the morphology of the WGM cavity[33]. After the geometries of the microcavities are defined, a reflow process is implemented to refine surface planarization. Figure 1(a) shows one silica microcavity with a diameter of 2.65 mm, and according to the zoomed-in image, a 318 µm radius of curvature is inferred. Using the finite-element method, the effective mode volume is computed for the silica microcavity with respect to the radius of curvature of its cross-sectional periphery. The effective mode volume of the fundamental transverse magnetic (TM) mode in the microcavity reaches . Studies have revealed a negative correlation between thermorefractive noise and the effective mode volume with the WGM cavities[34,35]. Furthermore, the larger the mode volume, the less pronounced the enhancement effect within the cavity, resulting in reduced levels of nonlinear effects. Therefore, strategic optimization of geometric morphology during cavity fabrication to appropriately enlarge the mode volume can effectively mitigate the cavity noise.
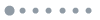
Figure 1.(a) Microcavity image and mode profile. (b) Schematic diagram of the microrod-taper coupling packaged structure.
To obtain maximum power coupling efficiency and ensure high Q resonances and long-term stable coupling conditions, we employ the heat-and-pull method[36] to fabricate the low insertion loss tapered fiber with a tapered region length of approximately 1 cm. Based on this, we propose an innovative scheme for packaging the coupling structure. The microrod-taper coupling packaged structure is outlined schematically in Fig. 1(b). The core component, an alloy casing with 3D printed polyetheretherketone (PEEK) substrate lining, features two perpendicular grooves for securing the silica microrod and tapered fiber. Circular apertures are incorporated at both ends of the shell to facilitate fiber passage and subsequent standardized end-to-end operation. Seal rings and silicone rubber are adopted for the isolation of water vapor and atmospheric particles, ensuring the hermetic seal of encapsulated microcavities. The microcavity is affixed within the peek liner using UV glue. Then, the six-axis precision displacement stage and custom fiber clamps are employed to fine-tune the coupling between the tapered fiber and the microcavity. Ultimately, the tapered fiber is immobilized using the multilayer adhesive with varying Young ’s modulus gradients and low thermal expansion coefficients. To maintain optimal coupling conditions during the curing of the adhesive, a vertically oriented circular ultraviolet radiation technique is employed to achieve uniform exposure and facilitate the optimized adhesion among the cavity, tapered fiber, and substrate. To further improve the spectrum stability of the device, we have implemented a proportional integral derivative (PID) temperature control system to prevent resonant position shifts and standardized FC/APC polarization-maintaining fiber connectors to select the mode for specific experiments. The negative temperature coefficient (NTC) thermistor and thermo-electric cooler (TEC) are utilized for precise temperature monitoring and regulation of the WGM cavity, which can be stabilized within the range of 5 mK. This packaging method strengthens the WGM microcavities’ immunity to temperature fluctuations, external vibrations, airflow, and surface contamination.
3. Characteristics of the Packaged Microcavities
To determine the Q factors of the microcavities, a measurement setup was constructed as depicted in Fig. 2(a). Light from a tunable laser (NKT, Koheras BASIK Module E15) with a linewidth of 0.1 kHz was launched into the tapered fiber to excite the microcavities. Note that the laser power is regulated by the attenuator to be less than 50 µW to suppress thermal effects. The Q factor is tested by characterizing the resonant mode linewidth. Figure 2(b) compares the Lorentz fitting spectrum before and after packaging for the same resonant mode of one cavity. The resonant dips exhibit minimal broadening after packaging, indicating the effectiveness of our packaging method in preserving the mode structure. Consequently, a packaged microcavity with an impressive Q factor of up to magnitudes is achieved. To elaborate, the coupling state of the microcavity remains largely consistent before and after packaging as a result of the lower curing shrinkage and smaller thermal expansion coefficient of the adhesive layers. Furthermore, the utilization of polarization-maintaining fibers allows for the selection of particular polarization states. By documenting the attributes of different mode families within one FSR, it can be guaranteed that the identical mode is being examined both pre- and post-encapsulation. As shown in Fig. 2(c), by extracting the maxima and minima from the ringdown signal with the packaged microcavity, the envelope of the oscillations indicates an exponential field decay from the resonator with a time constant of 3.20 and 3.69 µs, corresponding to a loaded Q factor of and , respectively. Figure 2(d) depicts the homogeneity of packaged microcavities. Five silica microcavities with a diameter of 2.65 mm were fabricated using identical parameters on a single microrod, as shown in the inset of Fig. 2(d). Subsequently, these microrods were packaged according to the procedure introduced above. The results presented in Fig. 2(d) demonstrate that our packaged microcavities exhibit excellent consistency in performance parameters (Q factor deviation , FSR deviation ). Such satisfactory preparation uniformity makes it promising for large-scale manufacturing.
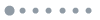
Figure 2.Experimental setup and results. (a) Schematic diagram of the WGM excitation and collection experiment setup. PC, polarization controller; PD, photodetector; OSA, optical spectrum analyzer; FG, function generator; OSC, oscilloscope. (b) Comparison diagram of the same typical resonant mode in the Q factor for unpackaged (left) and packaged (right) microcavities. The gray scatter in the diagram is the collected resonant mode waveform, and the red curve is the Lorentz fitting waveform. FWHM, full width at half-maximum. (c) Ringdown experiment of microcavity devices. The fitted exponential decay time (τ) are 3.20 and 3.69 µs corresponding to loaded Q factors of 1.95 × 109 and 2.25 × 109 , respectively. (d) The Q factors and FSR consistency of packaged microcavities. Inset: microscope images of five silica microcavities.
To verify whether the packaged microcavity devices are capable of properly operating in practical application scenarios, rigorous experiments were conducted in multiple dimensions, containing outdoor environments, vibration, and temperature shock experiments to simulate the interference of uncontrollable factors, which may be encountered during routine use, transportation, and storage processes. We conducted durability tests on both unpackaged and packaged silica microcavities in non-clean indoor conditions, simultaneously monitoring changes in the Q factors of the microcavities over time. For the facilitation of Q factor tracking, the unpackaged microcavity was attached to the tapered fiber using UV adhesive, yet it was not subjected to thermostatic isolation and sealed packaging for thermal and vibrational stability. Figure 3(a) shows that the Q factor of unpackaged microcavity degrades seriously within 4 days and cannot meet the requirements of microcomb application, while the Q factor of the packaged microcavity is not impacted. The condensation of water and deposition of microdust particles on the surface of the unpackaged microcavity when it is exposed to air contribute to the increased cavity surface loss, consequently leading to a significant reduction in the Q factor. Immediately after, we position the packaged microcavity in outdoor environments with uncontrolled temperature and humidity. As shown in Fig. 3(b), it is evident that the Q factors of the packaged microcavities do not exhibit any significant deterioration and consistently exceed , despite considerable fluctuations in ambient temperature and humidity during outdoor exposure. Besides, the packaged microcavity was pegged on the vibration apparatus for constant and variable frequency vibration tests. Three-axis vibration was set up with an amplitude of around 1.5 mm and an acceleration range from 0.28 to in the longitudinal, horizontal, and vertical directions. The vibration time at each fixed vibration frequency point was set as 6 h. Upon the completion of each 6 h fixed-frequency vibration experiment, the Q factor was assessed and meticulously documented, followed by the continuation of the subsequent phase of vibration testing, with frequencies tested in the sequence of 5, 50, 100, 200, and 600 Hz. The results plotted in Fig. 3(c) manifest the variation in the Q factors and normalized transmission of the optical microcavities with the vibration frequency. We can see that the Q factors of the microcavity do not decrease notably, and the normalized transmission can still be maintained above 90%. Additionally, a 6 h frequency-varying (0–600 Hz) vibration test was performed. The results manifest that the packaged microcavity still retains a high Q factor () and transmission ( ). To further bench the performance of the packaged microcavity, we tested the bearable thermal concussion for the packaged structure using a temperature chamber. The temperatures were set to 50, 0, 60, , 70, and successively to simulate the severe thermal condition not involved in outdoor exposure, and the data were recorded after 16 h of exposure at each temperature point in the sequence. From Fig. 3(d), it is clear that the Q factors and transmission of the microcavity device are scarcely impacted by substantial temperature fluctuation. It is imperative to note that the temperature limits for the package structure are to 90°C. Exceeding this temperature range can induce variations in the microcavity coupling state due to thermal expansion and contraction of the adhesive layer, resulting in a degradation of the Q factor magnitude. These serve as sufficient evidence which reveal that such a sealed structure effectively safeguards the coupling structure against external intrusion attributed to its superior resistance to thermal-induced microdeformation and ambient vibration, thereby making our packaged microcavity a robust device potentially suitable for use in a plurality of harsh environments.
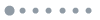
Figure 3.Robust experiment. (a) Comparison diagram of the Q factors of unpackaged and packaged microcavities placed indoors. (b) Variation of the Q factors of packaged microcavities placed outdoors. The red and black dashed lines represent the maximum and minimum temperatures, respectively, and the time points with high humidity have been marked in the figure. (c) Tested Q and normalized transmission of packaged microcavities after vibration experiments. The vibration frequency is marked next to each data point. (d) Tested Q and normalized transmission of packaged microcavities after temperature shock experiments. The blue and red dotted lines represent the changes of Q factors and normalized transmission, respectively. The testing temperature change rate is 10 K/min, and the above figure is approximately a sudden temperature change. Note that the improvement in the Q factor and transmission can be attributed to the coupling state ’s slight variations and measurement errors.
4. Soliton Microcomb Generation
To further characterize the performance of single-soliton Kerr frequency combs generated from our packaged microcavities, a tunable laser is employed to pump the specific resonant frequency in the negative dispersion region, and the laser is locked to a specific soliton power through the servo system[37]. The threshold power for parametric oscillation can be estimated according to the following theoretical formula[38]: where is the effective mode area, is the resonant frequency, and are, respectively, the linear and nonlinear refractive indices of the material, is the FSR in rad/s units, and is the coupling coefficient, with being the loaded Q factor. The threshold behavior was investigated experimentally by measuring the power of generated optical sidebands at various pump power levels. When the power of input light increased to 4 mW, the comb power shows an obvious soliton step displayed in Fig. 4(a) as the pump tunes red relative to the resonance, which verifies that the high Q factor acts as a pivotal part in threshold power reduction, exactly as predicted by the above formula. The optical spectrum of the soliton is illustrated in Fig. 4(b), which features a characteristic spectral envelope. The deviation from the theoretical curve is deemed to be the result of the dispersion induced by mode-crossing and the coupling strength[20]. The coherent nature of the soliton microcomb is confirmed by the photodetection of the soliton pulse streams. The 24.98 GHz repetition beatnote with high signal-to-noise ratio (SNR) is shown in Fig. 4(c). Compared with unpackaged optical microcavities, packaged microcavity devices exhibit reduced susceptibility to environmental perturbations due to the shielding of the vibration isolation structure and high thermal resistance lining, resulting in improved waveguide-cavity coupling stability and minimized resonant frequency drift. Therefore, we are able to maintain the soliton state stable for a longer time after being locked and more easily access the “quiet point” (QP) by modulating the pump-cavity detunings experimentally[25]. At the QP state, the comb repetition frequency is most insensitive to the detuning alteration, and the phase noise of the pump laser reaches the minimum resulting from the balance between the Raman effect and dispersion; thus, an additional 20 dB noise reduction can be obtained. Meanwhile, the betterment of the thermodynamic and mechanical robustness of the packaged device is conducive to reducing the phase noise of the soliton microwave oscillators, especially in the middle and low frequency bands. The typical phase noise levels of the soliton microcomb are characterized using a phase noise analyzer, which is plotted in Fig. 4(d). The low-frequency noise peaks caused by ambient perturbations are suppressed in the packaged soliton cavity. The resulting phase noises at the “quiet point” reach and at 1 and 10 kHz offset frequencies, respectively. It should be complemented that the small bump around 40 kHz next to the main peak of the repetition rate in Fig. 4(c) and the phase noise protrusion around 1 MHz in Fig. 4(d) dominantly stem from the pump laser and the erbium-doped fiber amplifier (EDFA), which is used to amplify soliton comb power before coupling into the photodetector (PD) and phase noise analyzer (PNA).
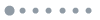
Figure 4.(a) Measured evolution of comb power pumped as the laser frequency scans across the mode from the blue-detuned to the red-detuned regions. The step in the red-detuned region is a characteristic feature of soliton formation. (b) Optical spectrum of the soliton microcomb. (c) Radio frequency (RF) spectrum showing the soliton repetition rate. (d) Measured single sideband (SSB) phase noises before and after packaging at three different laser-cavity detunings.
5. Conclusion
In summary, we have demonstrated a fabrication and packaging scheme that endows our devices with ultra-high Q factors, appreciable robustness, compactness, and homogeneity. The rigorous characterization experiments were performed and showed that the packaged microcavity would potentially benefit many applications under sophisticated conditions. We further observe the single-soliton Kerr frequency comb with relatively low pump power, exhibiting more appealing noise performance than the non-packaged one. This work provides a feasible solution for the simply and robustly assembled package technique for discrete optical microcavities as an ideal photonic building-block applicable to soliton light sources and other fields including external-cavity narrow linewidth lasers[39,40] and ultra-narrow optical filters[41], with the superiority of absorption cost reduction, system stability upgradation, and batch production. We believe that our efforts illuminate a pathway toward the broad range of optoelectronics applications of optical microcavities in aerospace, navigation, and vehicular systems. Meanwhile, our efforts also offer a valuable guideline for specialized packaging in other platforms such as optical precision sensing [42–44] and coherent control[45, 46].