1 Introduction
Due to the characteristics of easy deployment, low maintenance cost, high mobility, and strong hovering ability of drones, the unmanned aerial vehicles (UAVs) application areas are becoming increasingly widespread, e.g., the real-time monitoring, providing wireless coverage, remote sensing, search and rescue, cargo delivery, security and monitoring, precision agriculture, and civilian infrastructure inspections [1–3]. As the front-end of the UAV communication system, the airborne antenna determines the quality of the entire wireless communication system.
For the design of antenna systems on UAV platforms, the following four aspects should be considered: 1) due to the limitations of the drone’s own payload, the smaller the size and weight of the antenna, the more competitive it becomes, while the antenna meets the radiation performance requirements; 2) usually, UAV platforms are not flat but curved, and further consideration can be given to the conformal problem of the antenna system involved with the platform; 3) it is necessary to consider impedance matching for multi-antenna systems on airborne platforms, which requires simultaneously meeting the impedance matching performance of each antenna element in specific environments; 4) within a limited size, the isolation performance of each antenna port is a key factor to consider. Therefore, the airborne antenna should be small in size, light in weight, thin in thickness, and conform to the body. At the same time, the impact of the antenna structure on the flight performance of UAV should also be considered.
According to the characteristics of drone flight, the directional patterns of airborne communication antennas should exhibit omnidirectionality on the horizontal plane to avoid communication blind spots between drones and base stations. Common antenna types include spiral antennas [4], inverted F antennas [5], single cone antennas [6], dipole antennas [7], and knife shaped antennas [8]. Due to the multiple application scenarios of drones, airborne antennas are often demanded to have dual-band or multi-band characteristics [9–11]. The above antennas are all single antennas based on drone platforms, which have certain shortcomings in gain, pattern control, and other aspects. In order to improve the performance of a single antenna, a multi-antenna system is designed, including array antennas [12–14] and multiple input multiple output (MIMO) antennas [8,15,16]. Unlike array antennas that focus on pattern control, MIMO antennas are mainly applied to increase the channel capacity of wireless communication systems and improve transmission rates and reliability. Reference [8] designs a 2-unit MIMO knife shaped antenna consisting of monopole and dipole antenna units, both of which operate in the 2.4 GHz frequency band. Reference [15] designs a 3-unit dual-mode MIMO antenna system integrated on a drone platform, with each unit operating simultaneously in the 2.4 GHz and 5.2 GHz frequency bands. Reference [16] presentes a compact ultra-wideband MIMO slot antenna with band notch characteristics for portable wireless ultra-wideband (UWB) applications. A Minkowski fractal parasitic stub along with a Minkowski fractal grounded stub was placed at 45° between the monopoles to reduce the coupling between them which in turn establish high isolation between the radiators. The results illustrated improving isolation using compact fractal structures which occupy less space than conventional isolation mechanisms in MIMO structures. Compared with traditional antennas, multi-antenna systems have higher data transmission rates and longer transmission distances, which is beneficial for improving the communication performance of drones. To the best of the authors’ knowledge, there is currently no literature reporting on the integration of multiple antennas with different operating frequencies for drone platforms.
This article designs a dual-band airborne composite antenna, which includes two types of antennas operating in the S-band (2.2 GHz–2.4 GHz) and the 5G band (4.8 GHz–4.9 GHz), both of which are vertically polarized and shared-aperture. The S-band antenna is composed of a rectangular monopole antenna, while the 5G band antenna is composed of four element monopole MIMO antennas. In addition, a dual open loop MTM structure is introduced to improve the isolation between antenna elements.
2 Antenna design
2.1 Overall structure of the airborne antenna
As shown in Fig. 1 (a), the dual-band airborne antenna consists of an S-band monopole antenna (A1) and a 5G band 4-element monopole MIMO antenna (A2, A3, A4, and A5), which are all perpendicular to a circular metal ground plate with a thickness of 3 mm and a radius Rd of 150 mm. To improve the isolation between antennas and balance the miniaturization of the antenna size, 6 double open loop MTM structures were inserted between different antenna elements. In Figs. 1 (b) and (c), it can be observed that all antenna radiation elements are located on the front side of the substrate, while the double open loop structure is placed on the back of the substrate. In order to ensure the connection between the antenna and the ground plane during processing and assembly, a metal strip with the length of 124 mm and a height and width of 3 mm is added below the back of the antenna substrate as shown in the shaded area in Fig. 1 (c). Note that the antenna substrate uses a double-sided Taconic TRF-45 board with the thickness of 1.016 mm, the relative dielectric constant of 4.5, and the loss tangent of 0.0035. Then, the proposed dual-band and high-isolation shared-aperture antenna is simulated and optimized by the commercial software High Frequency Structure Simulator (HFSS), and its optimized dimensions are marked in Fig. 1.
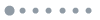
Figure 1.Schematic diagram of airborne composite antenna structure: (a) perspective view of antenna, (b) front view of antenna, and (c) back view of antenna (unit: mm).
2.2 Design of S-band monopole antenna
In Fig. 1 (a), the S-band monopole antenna A1 consists of a rectangular radiation patch, a 50-Ω microstrip feed line, and a rectangular transition between the microstrip and the radiation patch. To illustrate the performance of the monopole antenna, the monopole MIMO antenna and the double open loop structure in the 5G frequency band are removed, while the remaining structures are unchanged. Fig. 2 shows the reflection coefficient of the monopole antenna. It can be observed that the center frequency of the antenna is 2.3 GHz, and its reflection coefficient is below –24 dB in the range from 2.2 GHz to 2.4 GHz. Besides, Fig. 3 displays the two-dimensional radiation pattern in the xy-plane, which is omnidirectional radiation in that plane.
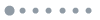
Figure 2.S-parameter of the S-band antenna.
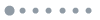
Figure 3.2D radiation pattern of the S-band antenna in the xy-plane.
2.3 5G frequency band MIMO antenna design
In the 5G frequency band, the 4-element monopole MIMO antenna is shown in A2 to A5 of Fig. 1. This MIMO antenna consists of a rectangular radiation surface and a 50-Ω microstrip line. In order to investigate the characteristics of the 5G antenna, only the antenna A2 of Fig. 1 is retained, and the other antennas, i.e., A3, A4, and A5, and the open loop structures of Fig. 1 are removed. As seen in Fig. 4, the simulation results of the antenna A2 show that the reflection coefficient is below –24 dB in the frequency range from 4.8 GHz to 4.9 GHz.
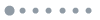
Figure 4.S-parameter of the 5G band antenna.
2.4 Isolation improvement analysis of antenna elements by using MTM structures
Two decoupling methods are commonly used for MTM MIMO antennas. One is to load MTMs in the same plane as the antenna to improve isolation [17], and the other is to load MTMs perpendicular to the antenna to improve isolation [18]. This design chooses the former, i.e., placing a double open loop MTM structure in a flat manner. In order to elucidate the effect of the MTM structure on the isolation of antenna elements, Fig. 5 compares the isolation between antenna elements with and without the MTM structure. Fig. 5 (a) shows the isolation between the 5G band MIMO antenna element and the S-band monopole antenna. Without the MTM structure, the isolation between A1 and A4 is the worst, which is about –17.2 dB in the 4.8 GHz to 4.9 GHz frequency band. After adding the MTM structure, the isolation between antennas is significantly improved, below –25 dB in the above frequency band. Fig. 5 (b) displays the isolation between MIMO antenna elements in the 5G frequency band. If the MTM structure is not added, the antenna elements A2, A3, A4, and A5 have poor isolation due to their proximity, with a minimum of –9.5 dB in the 4.8 GHz to 4.9 GHz frequency band. However, their isolation degree is all below –20 dB, when the MTM structure is added. Besides, Fig. 6 compares the current distributions between antenna elements with and without the MTM structure. Without the MTM structure, it can be found that strong currents are coupled from port 2 to port 3, resulting in poor isolation between A2 and A3. With the MTM structure, there is almost no coupled current, and the current is confined near the antenna which improves the isolation between A2 and A3. It demonstrates that the isolation between antenna elements can be improved by using the MTM structure.
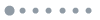
Figure 5.Effect of the MTM structure on the isolation between antenna elements: (a) isolation between S-band and 5G band MIMO antenna elements and (b) isolation between 5G band MIMO antenna elements.
Next, taking the MIMO system’s antenna elements A2 and A3 as an example to explain the decoupling principle, other antenna elements are similar. Without the MTM structure in the antenna, the energy of the excited antenna A2 is mainly coupled to the antenna A3. Due to the different propagation paths of electromagnetic waves, the energy coupled to A3 is mainly divided into two parts. One is spatially coupled to A3, i.e., the spatially coupled. The other part couples with the surface medium to A3, i.e., dielectric coupling. Because of the strong coupling effect between the two antennas, it is easy to cause a sharp deterioration in the radiation performance of the antennas. When the MTM structure is added between the antennas A2 and A3, the isolation between MIMO antenna elements will increase. Two reasons for this phenomenon are considered here. On the one hand, the electromagnetic MTM structural units loaded on the same layer also participate in radiation and radiate the energy opposite to the radiation direction from A2 to A3. The radiated energy can cancel out some of the coupling energy and greatly reduce the formation of spatial coupling waves. On the other hand, the presence of the MTM structure can reduce the coupling of surface waves, which can suppress the coupling effect between antennas.
In addition, the antenna spacing also has an impact on the isolation. However, given the limited space of the drone platform, the isolation performance between each antenna element within the limited system size is addressed here. In actuality, the isolation between antenna elements will be further improved when the drone platform size increases, leading to an increase in the spacing between the MIMO antennas.
2.5 Influence of ground plane size on antenna performance
In the airborne fusion antenna system depicted in Fig. 1, the antenna prototype is designed, constructed, and tested using a circular ground with the radius of Rd = 150 mm. When the antenna is mounted on a drone platform, the drone’s body can be considered as the ground plane of the antenna. Therefore, in order to ensure the performance of the antenna, the ground plane size should have a relatively small impact on the frequency of the antenna. Figs. 7 (a) and (b) show the influence of ground plane size on the reflection coefficients of the S-band antenna and the 5G band antennas A2 and A3. We can observe that when the radius of the ground plane varies from 120 mm to 180 mm, the reflection coefficient of the antenna does not change significantly. This indicates that there is no significant frequency offset when working on different ground plane sizes, thus ensuring the performance of the multi-antenna system on the airborne platform.
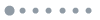
Figure 6.Comparison of the current distribution of MIMO antenna at 4.85 GHz: (a) without and (b) with open loop.
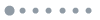
Figure 7.Effect of the ground plane radius on MIMO antenna performance: (a) S-band antenna and (b) 5G antenna elements A2 and A3.
3 Measurement and analysis of airborne composite antennas
In order to verify the performance of the proposed airborne antenna, the antenna prototype is constructed and shown in Fig. 8.
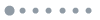
Figure 8.Photos of the fabricated antenna: (a) front view, (b) back view, and (c) measurement.
3.1 Reflection coefficient and isolation
In the first step, the reflection coefficient of the S-band monopole antenna was tested by using the Rohde & Schwarz ZVB20 vector network analyzer and compared with the simulation results. As shown in Fig. 9, the tested –10 dB bandwidth of 1.92 GHz to 2.75 GHz is slightly wider than the simulated bandwidth of 1.94 GHz to 2.74 GHz. Then, the S-parameters of the monopole MIMO antenna elements in the 5G frequency band were tested. Fig. 10 (a) compares the reflection coefficient of port 2 to port 5. It can be found that the common –6 dB impedance bandwidth is 4.75 GHz to 4.91 GHz, which can cover the 5 GHz frequency band (4.8 GHz to 4.9 GHz). In addition, Fig. 10 (b) shows the representative isolation between MIMO antenna elements in the 5G frequency band, and the isolation is all better than 23 dB. Fig. 10 (c) gives the isolation between the MIMO antenna and the S-band antenna, and their isolation is all better than 21 dB. Moreover, the test results are basically consistent with the simulation results. Therefore, it can be demonstrated that the isolation between each antenna is good.
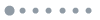
Figure 9.Reflection coefficient of the S-band antenna.
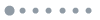
Figure 10.S-parameters of the MIMO antennas in the 5G frequency band: (a) reflection coefficient of MIMO antenna elements, (b) isolation between MIMO antenna elements, and (c) isolation between S-band antenna and 5G band MIMO antenna elements.
3.2 Radiation performance
In addition, the radiation pattern of the antenna was tested in the Satimo microwave anechoic chamber, as shown in Fig. 8 (c). Fig. 11 shows the radiation pattern of the S-band monopole antenna in the xy-plane, which exhibits omnidirectional radiation characteristics, and the measured and simulated patterns have good consistency. Besides, the three-dimensional radiation pattern of each antenna in the 5G frequency band at 4.85 GHz is also presented in Fig. 12. Due to the electromagnetic coupling effect between antenna elements and the existence of a double open loop structure, the omnidirectional radiation performance of each antenna element will be affected.
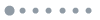
Figure 11.Radiation pattern of the S-band antenna in the xy-plane at 2.3 GHz.
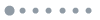
Figure 12.Radiation pattern of MIMO antenna at 4.85 GHz: (a) antenna A2, (b) antenna A3, (c) antenna A4, and (d) antenna A5.
3.3 Envelope correlation coefficient
The envelope correlation coefficient (ECC) is an important parameter that characterizes the degree of correlation between various channels in MIMO antenna arrays, which affects the transmission rate of signals and the diversity performance of the system. ECC between antenna elements in MIMO arrays can be calculated by [19]
$ {\text{ECC}} = {\rho _{ei{\mathrm{,}}j}} = \frac{{\left| \displaystyle\oiint
{({A_{i{\mathrm{,}}j}}(\Omega ))} {\text{d}}\Omega \right|^2}}{{ \displaystyle\oiint
{({A_{i{\mathrm{,}}i}}(\Omega ))} {\text{d}}\Omega \cdot \displaystyle\oiint
{({A_{j{\mathrm{,}}j}}(\Omega ))} {\text{d}}\Omega }}$ (1)
where $ {A_{i{\mathrm{,}}j}}(\Omega ) = {\text{XPR}} \cdot {E_{\theta i}}(\Omega )E_{\theta j}^*(\Omega ){P_\theta }(\Omega ) + {E_{\varphi i}}(\Omega )E_{\varphi j}^*(\Omega ) {P_\varphi }(\Omega ) $. i, j=1, 2, 3, 4 are the port numbers for the MIMO antenna element, and the XPR is the cross polarization ratio. $ {E_\theta }(\Omega ) $ and $ {E_\varphi }(\Omega ) $ are the $ \theta $ and $ \varphi $ components of the radiated electric field, respectively. $ {P_\theta }(\Omega ) $ and $ {P_\varphi }(\Omega ) $ represent the $ \theta $ and $ \varphi $ components of the incident wave angular density function, respectively. $ \Omega $ is the solid angle. $ {A_{i{\mathrm{,}}j}}(\Omega ) $ can be obtained from the simulation software.
Fig. 13 shows the ECC curves of the antenna elements A1 and A2 (ECC12), A2 and A3 (ECC23), and A2 and A4 (ECC24). Obviously, within the operating frequency range from 4.8 GHz to 4.9 GHz, the maximum simulated ECC values are all below 0.02, far below the threshold of 0.5 required for MIMO systems. This indicates that the proposed MIMO antenna array has good MIMO diversity performance in the system.
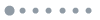
Figure 13.Envelope correlation coefficient of the antenna.
4 Conclusions
This paper presents a multi-antenna, dual-band, high-isolation, and shared-aperture airborne antenna system, which consists of an S-band monopole antenna and a 5G band MIMO antenna. The associated measured and simulated results show that: 1) the S-band antenna exhibits omnidirectional radiation characteristics; 2) by adding some double open loop MTM structures between antenna elements, the isolation between them can be improved remarkably. Specifically, the isolation between the 5G band MIMO antenna element and the S-band monopole antenna is better than 21 dB, while the isolation between the MIMO antenna elements is better than 23 dB; 3) the ground size has no significant effect on the antenna operating frequency, which indicates that the antennas can be mounted on airborne platforms of different sizes.
Therefore, it can be demonstrated that the proposed antenna has a wide bandwidth, high isolation, good radiation, and diversity performance. It indicates that the proposed dual-band and high-isolation shared-aperture antenna is a good candidate for airborne UAV systems and other aircraft platforms. More details about the application of the dual-band shared aperture antenna for the UAV platforms or similar airborne platforms can refer to Refs. [10], [20], and [21].
Disclosures
The authors declare no conflicts of interest.