Introduction
Amongst the three groups of essential biomaterials, fats, sugars and proteins, proteins are the most vital in cell development, cell-to-cell communication and cellular sensing
1
,
2
. As such, the selective binding of proteins is an attractive means of performing various analytical tests and of achieving targeted drug delivery. Furthermore, the formation of three-dimensional (3D) protein microenvironments that can control or influence cellular growth and development is currently of significant interest in the field of tissue engineering.
Based on
in situ
gelation processes, protein hydrogels have been fabricated by solvent exchange, ultraviolet (UV) irradiation, ionic cross-linking, pH changes and temperature modulation
3
. The use of enzymes can also create artificial, cross-linked hydrogels with free enzyme
4
, localized
5
or self-assembled structures
6
. These methods allow the formation of complex nano-scale protein hydrogel structures with high-throughput in a controlled manner
4
. Cell-laden cross-linking using a photoinitiator in conjunction with UV exposure can lead to cytotoxicity, while physical cross-linking results in lower mechanical stability compared to a covalently bonded hydrogel
4
. Nevertheless, because the cross-linking density, resulting mechanical properties and applicable residues are all well controlled by these methods, microfluidic mixers
7
,
8
, drug delivery colloids
9
and rare element mining
10
are promising applications, in addition to cell culturing for tissue engineering and regenerative medicine
4
,
8
. These techniques can produce large quantities of structures at reasonable speeds, although it is challenging to establish suitable microenvironments for biological studies.
Since microfluidic devices can create shell-and-core streams (so-called laminar flow) in microchannels, shell-and-core fibers containing protein and matrix material are fabricated at desired diameter and length
11
. Cells can be suspended into a desired stream and grown inside the specific environment
11
. Dissolvable materials grown in the outer shell can be used to support handling and transport of these fibers as well as to prevent bacterial infection
11
. Cellular structures that prefer elongated environments are more readily obtained using such configurations
12
,
13
. However, limited growth space and difficulty in the spatial patterning of cells in the case of certain flow processes represent current challenges associated with this technology.
Rather than using laminar flow to shape a microenvironment, proteinaceous microspheres can be obtained from water-in-oil emulsions
14
or by high-intensity ultrasound
15
. The size distribution and dispersion of these microspheres can be fine-tuned by varying certain parameters and additives
14
. In the case of the ultrasound method, either air of liquids other than water can be employed
15
. In addition, because proteins are not significantly denatured within nanometer-sized shells
15
, these microspheres can be applied to wound healing in association with appropriate oils
16
. In another application, microbubbles filled with sonographic contrast agents were applied to contrast-specific imaging in animals based on non-linear optical interactions
17
. Fabrication by emulsion or ultrasound allows processing that is both rapid and large-scale, although the resulting spherical structure size distributions are limited.
In order to mimic the complexity of biological systems using artificial proteinaceous structures, a more diverse selection of protein microenvironments is required, along with increased spatial resolution. Ink jet printing based on gel droplets has been utilized to obtain improved spatial control, 3D fabrication capabilities and the ability to create multi-material and multi-cellular hydrogels
18
. Although this process involves the release of cell-laden or bare protein matrix droplets and is time consuming, it permits the engineering of complex microenvironments within a given volume
19
,
20
. Using sacrificial materials, it is also possible to create hollow features, such as vascularized tissues
21
. The extent of spatial control is, however, limited to the nozzle aperture size and can also be restricted by the associated gelation processes, such that the typical fabrication resolution is currently several tens of μm. Additionally, soft structures obtained using this method are often difficult to move or handle during subsequent processing or cell culture analysis steps.
Using laser light as a propulsion source, proteins and cells can be transferred from a thin-film biomaterial deposited on a transparent donor substrate to the surface of an accepter substrate
22
. This process is termed laser-induced forward transfer (LIFT). Modulating the impact strength and the number of stacked layers can produce thick 2D structures, and even 3D structures become feasible by the use of sacrificial materials. Similar to an inkjet process, this transfer method requires no contact by the fabrication device and so the fluid properties are not a primary factor. Unfortunately, the requirement to propel the film material to another surface limits throughput, since the layer thickness achieved by a single laser shot is limited to several hundreds of nanometers
23
. Thicker cell-laden hydrogel layers have been patterned by taking advantage of the thermal expansion of a polyimide donor plate or based on absorption by a metal layer formed between the biomaterial thin film and the donor substrate
24
,
25
. Although the resolution of this method (at approximately several μm) is superior to that obtained using an inkjet process, the formation of complex 3D structures is difficult.
Recently, the photo-induced cross-linking of proteins using laser direct writing has attracted much attention as an alternative means of producing 3D proteinaceous micro- and nano-structures with high resolution.
Figure 1
shows a general setup and a schematic description of the fabrication of proteinaceous microstructures and will be discussed in greater detail later. In such processes, solutions containing mixtures of proteins and photoinitiators (or photosensitizers) are used as precursors
26
. The multiphoton cross-linking of proteins is similar to multiphoton polymerization using photocurable resins or negative-tone resists. This mechanism has also been employed for the cross-linking of DNA for cell analysis
27
,
28
. Utilizing a femtosecond (fs) laser multi-photon technique has further enabled the creation of complex 3D structures
29
,
30
. In multi-photon cross-linking, multiple spatiotemporally coincident longer wavelength photons generate an absorption scheme equivalent to that of a single photon having a shorter wavelength, in a process known as multiphoton absorption. As a result, electrons in materials can be excited solely at a focal volume of incident laser light, at which photon intensity exceeds the threshold intensity to substantially satisfy the probability of multiphoton absorption. Thus, photo-induced cross- linking can be confined to the focal volume. Furthermore, since the beam profile is Gaussian and the probability of multiphoton absorption is proportional to the laser intensity raised to the power of
n
, where
n
is the number of photons absorbed, the effective spot size will be smaller by the factor 1/
n1/2
than the spot size for single photon absorption
30
,
31
, enabling spatially localized cross-linking with higher resolution.
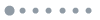
Figure 1.Laser writing system for microfabrication of proteinaceous structures.(a) Laser writing system is composed of three basic components: a laser, a lens and mirror system, and an objective lens. The beam is directed towards the sample mounted on a scanning stage, optionally observed with a white light camera setup. The system is controlled by a computer. The laser beam typically has a spatially Gaussian profile. In a plane perpendicular to the laser beam axis, the cross-linking occurs only in the beam where the intensity exceeds a threshold that is dependent on wavelength, absorption scheme (single or multiphoton absorption) and the protein precursor. (b) In volume, protein cross-linking occurs in the laser focus along the writing path in the protein precursor material. Diameter and height of volume elements, so-called voxel, in a free space become ellipsoidal shapes due to mismatch between focused spot sizes and Rayleigh length, as indicated by dashed and solid lines. On the substrate, surface bound structures are formed, depending on the position of focus. Determining resolution as the smallest distance between distinguishable structures, well-connected 3D structures can be formed by scanning the focused laser beam with a step smaller than the resolution. The box inset shows that protein molecules (light blue) in the precursor are interacted with photoactivator (orange) to be cross-linked (dark blue). The circular inset shows an SEM image of an icosahedron with submicron feature sizes, scale bar represents 1 μm, reprinted from ref. 33, with the permission of AIP Publishing, copyright 2015.
Based on the absorption properties of the precursor solution (the mixture of proteins and a photoinitiator or photosensitizer), an appropriate femtosecond laser wavelength must be selected to restrict the solidification process solely to the focal volume during the multi-photon process
32
. The non-invasive nature of forming cross- linked proteins in solution enables the fabrication of various complex embedded 3D structures. These structures can be nested on the substrate surfaces
33
,
34
or embedded into transparent devices such as microfluidic channels
35
-
37
.
In addition to 3D fabrication and superior resolution, another advantage of the multi-photon cross-linking of proteins as 3D printing materials is the potential to retain the protein functions after fabrication
35
,
38
,
39
. These functions include not only the diverse intrinsic properties of native proteins such as charge transfer, molecule modification and cell-cell communication, but also engineered functions of synthetic proteins. Consequently, the resulting protein structures have been applied to fabricate functional microdevices, including pH-sensitive actuators
39
,
40
and optical microcomponents
41
,
42
. The integration of proteinaceous microstructures into commercial polymers
43
and protein-polymer hybrids
44
has also been found to increase mechanical strength.
This review article presents a comprehensive summary of the current understanding and recent advancements in the femtosecond laser cross-linking of 3D proteinaceous micro- and nano-structures. Protein types and fabrication conditions employed in previous works are summarized, and the possible mechanisms associated with this technique as well as the properties of the treated proteins are discussed. Advances, present-day applications and the potential for future applications are also described. Due to the abundance of native, modified and synthetic proteins with various functions, we believe that the femtosecond laser cross-linking of proteinaceous microstructures offers numerous applications in biological studies, lab-on-a-chip and total analysis systems.
Survey of previous research
Table 1
summarizes the studies reported to date regarding femtosecond laser cross-linking in terms of the protein solutions employed, while
Table 2
presents the laser fabrication conditions. In this section, both fabrication conditions and the properties of the resulting cross-linked proteins are discussed.

Table 1. Protein solution conditions.
A summary of previous fabrication conditions, focusing on protein and photosensitizer concentrations. Examples of typical solvents and protein databank samples are included. Photoactivator abbreviations: rose bengal (RB), methylene blue (MB), flavin mononucleotide (FMN), flavin dinucleotide (FAD), rhodamine B (RhB), rhodamine 6 G (R6G), benzophenone dimer (BPD), sodium 4-[2-(4-morpholino)benzoyl-2-dimethylamino]butylbenzenesulfonate (MBS). Solvent abbreviations are: dimethyl sulfoxide (DMSO), phosphate-buffered saline (PBS), 4-(2-hydroxyethyl)-1-piperazineethanesulfonic acid (HEPES).
Table 1. Protein solution conditions.
A summary of previous fabrication conditions, focusing on protein and photosensitizer concentrations. Examples of typical solvents and protein databank samples are included. Photoactivator abbreviations: rose bengal (RB), methylene blue (MB), flavin mononucleotide (FMN), flavin dinucleotide (FAD), rhodamine B (RhB), rhodamine 6 G (R6G), benzophenone dimer (BPD), sodium 4-[2-(4-morpholino)benzoyl-2-dimethylamino]butylbenzenesulfonate (MBS). Solvent abbreviations are: dimethyl sulfoxide (DMSO), phosphate-buffered saline (PBS), 4-(2-hydroxyethyl)-1-piperazineethanesulfonic acid (HEPES).
Representative PDB code | Protein (mg/mL) | Photoactivator (mM) | Solvent | References | 1B8J | Alkaline phosphatase (AP) | 280-350, 9800, 2100 | RB | 0.25 | Ascorbic acid (1 mM) | 38 | AP-BSA | 38
,
32 | 1JV5 | Antibody anti-A | n.a. | Eosin-Y | 0.3 | n.a. | 35 | 1VYO | Avidin | 50-400 | FMN, FAD, MB, RB | 0, 1.2-8.5 | Distilled water or buffer(PBS or HEPES), pH ~7.4 | 39
,
45
-
49 | Eosin-Y, MB | 14.3, 4.3 | DMSO 21% in HEPES buffer | 34 | FAD with RhB | 0.250 with 0.407 | 12.5% HEPES, 19% DMSO, organic solvents | 43 | 4F5S | Bovine serum albumin (BSA) | 50-600, 800-1000
53 | Eosin-Y, Texas-Red, RhB, MBS, R6G, BPD, RB, FMN, FAD, MB | 0-100 | Distilled water or buffer (PBS or HEPES), pH ~7.3-4 | 32
,
35
,
37
,
39
,
41
-
42
,
44
-
61 | 210-460 | Eosin-Y, MB, RB, RhB with FAD | 4-14.3, 0.3-0.6 with 0.25 | 18%-23% DMSO in HEPES buffer (organic solvent) | 34
,
40
,
43
,
62
-
63
,
66 | 0-200 | FAD, Benzophenone- biotin | 0-2, 3-22 | 50% DMSO in buffer | 33
,
64 | 100, 200 | MBS | 67-100 | 50%wt glycerol-water | 37 | Biotinylated BSA (b-BSA) | 100-300 | FMN, FAD | 1-5 | Buffer (PBS or HEPES, pH ~7.4) | 48
,
61 | BSA conjugated with dye (dye-BSA) | 200-400 | RB, MB | 0, 0.25, 5 | Acetic or buffer, pH ~7.3-4 | 38
,
48
,
58
,
65 | 3HQV | Collagen type-Ⅰ(Col.Ⅰ) | 15, 30, 45 | FMN | 0.6, 1.2, 1.8 | Acetic acid, aqueous | 67 | Collagen type-Ⅰ, Ⅱ, or Ⅳ (Col.Ⅰ, Col.Ⅱ, Col.Ⅳ) | 2-3 | BPD, RB | 0.2, 1 | Acetic acid, aqueous | 52 | 1M3D | Col.Ⅳ - BSA | 0.15, 25 | FAD with RhB | 0.125 with 0.3 | mixed DMSO, HEPES buffer, organic solventAs purchased (pH=5) | 43
,
66 | 1GKB | ConcanavalinA (ConA) | 4.5 | RB, Texas-red, RhB | 0.5 | n.a. | 69 | 1OCC | Cytochrome c (CytC) | 100, 200 | FAD | 0, 4.5, 10 | Supporting electrolyte, pH=7.40. | 46
,
49
,
68 | 2Y0G | Enhanced green fluorescent protein (EGFP) | 2.35 | MBS | 10 | HEPES-KOH buffer, pH = 7.4 | 37 | 3GHG | Fibrinogen (FNG) | 3.9 or as purchased | RB, Texas-red, RhB | 0.01-0.1 | As purchased | 32
,
69 | 3M7P | Fibronectin (FN) | 1-1.29 | RB, Texas-red, RhB | 2-4 | Distilled water | 44
,
59
,
69 | 2CMM | Myoglobin (MGN) | 40 | RB, MB | 8.5 or 5 | HEPES buffer (pH 7.4) | 47 | 5IK4 | Laminin (LN) mixed with BSA | n.a. | FAD, MB | 1-4, 0.25 | HEPES buffer (pH 7.4) | 56 | 2CDS | Lysozyme (Lys) | 300-400 | MB, FAD | 1.2-5 | HEPES buffer (pH 7.4) | 45
,
47 | 320-400 | Eosin-Y, FAD, MB | 4.3-14.3 | DMSO 21%, HEPES buffer | 34
,
39 | 3UA0 | Regenerated silk fibroin (RSF) | 25 | MB | 5 | Aqueous, pH=7.0 | 70 | 1S0Q | Trypsin (TR) | 20 | FAD, Eosin-Y | 6, 0.3 | n.a. | 36 |
|

Table 2. Laser fabrication conditions.
Fabrication sorted by pulse width: femtosecond (fs), picosecond (ps) and nanosecond (ns). For almost all reports, high-NA lenses were used. BSA was employed for all conditions. Dynamic mirror device (DMD), spatial light modulator (SLM), or galvano mirrors were used in some of these previous works.
Table 2. Laser fabrication conditions.
Fabrication sorted by pulse width: femtosecond (fs), picosecond (ps) and nanosecond (ns). For almost all reports, high-NA lenses were used. BSA was employed for all conditions. Dynamic mirror device (DMD), spatial light modulator (SLM), or galvano mirrors were used in some of these previous works.
| Device | Pulse width | Frequency | Wavelength (nm) | Fabrication conditions | Special setups | Feature size | Protein | References | fs | Oscillator(Ti:Sa primarily) | < 29 fs, 100–120 fs, 400 fs | 54 MHz, 75 MHz, 76 MHz, 80 MHz, 82 MHz | 525, 715, 740, 750, 780, 800, 806, 850 | 20–123 mW, 3.385-6.093 mW, 0.5–7 µm/s, correcteddose equivalent K_L:0.01–100, 100–500pJ/pulse, single spot 1ms, 30–87 mW/μm
2 | | 1 μm at780 nm, 1.5 μmat 850nm | BSA, RSF, FN, CytC, TR, Avidin, b-BSA, AP, BSA-AP, dye-BSA, Col.Ⅰ, Col.Ⅱ, Col.Ⅳ | 36
-
38
,
42
,
44
-
45
,
48
,
50
,
52
,
55
,
59
,
61
,
68
-
70 | 100 fs, 120 fs | 76 MHz, 80 MHz | 740, 800 | 1.6 µs/pixel, 120 mW,
exposure doses 0–1.2
10
8
mJ/cm
2
, 1000 μs/ 100 nm/20–40 mW,
24–66 mW/μm
2 | galvano scan | 200 nm | BSA, FNG, FN, ConA, AP, Avidin, CytC | 32
,
41
,
46
,
51
,
53 | 60 fs, < 200 fs | 76 MHz, 80 MHz | 730–740, 750 | 5–105 mW, raster scan velocity of approx. 7 mm/s and a slow-axis velocity of 5–6 μm/s | DMD | submicron(0.5 μm) | BSA, dye-BSA, Avidin, Lys, MGN | 34
,
39
,
47
,
57
-
58
,
60
,
64 | Fiber | 94 fs, 100–200fs, 140 fs, 360 fs | 200 kHz, 79.8-80 MHz | 522, 740, 780, 790 | 0.09–144 mW, 0.2–10µm/s, 30 µm/s, 70µm/s, 2.4–9.1 nJ/µm2 energy density | | feature sizes > 250 nm, > 150 nm | BSA, BSA/LN, Col.Ⅰ, Col.Ⅳ, Avidin, EGFP | 33
,
37
,
40
,
43
,
56
,
62
-
63
,
66
,
67 | ps(subns) | Frequencydoubled μ-chip | 550 ps | 7 kHz | 532 | Corrected dose equivalent K_L:0.01–100 | | | BSA | 44 | Diodepumped Q-switched | 800 ps, 600 ps | 8 kHz, 7 kHz | 532 | Scanning up to 50μm/s, 277–321 GW cm
−2
peak, pulse E 3.5 µJ, 0.5–6 mW | | 140 nm(surface bound line) | BSA, CytC | 48
,
49 | Microlaser | 500 ps | 6.5 kHz, 40 kHz | 532 | 15, 20, 40 mW, 5 µm/s, 0.15 mW of laser power and 1 ms exposure time | | | BSA, TR, anti-A antibody | 35
,
36
,
50 | ns | Q-switched solid state | 12 ns | 20 kHz | 532 | 1 mW, 62 mW, 2–8 fps | SLM | | BSA, dye-BSA | 54
,
65 |
|
Fabrication conditions
Lasers and direct writing systems
In order to initiate cross-linking, a laser beam is applied to a precursor material. The beam path can be guided and shaped in various ways, and the overall system includes at least three basic components as shown in
Fig. 1a
: a laser, a lens and mirror system to direct and (optionally) shape the light, and an objective lens to focus the laser beam on the sample.
The laser beam, typically a linearly polarized Gaussian beam, is expanded by a collimation system to match the back-end aperture of the objective lens. Static mirrors are the simplest optical element used to guide the laser beam towards the sample. The average power of the laser can be controlled by rotating a half waveplate placed in front of a polarizer. If the combination of the half waveplate and the polarizer does not allow sufficient attenuation of the femtosecond laser pulses because of the limited extinction ratio, additional neutral density filters can be inserted after the polarizer to obtain a broader tunable range. The laser beam focused by the objective lens subsequently irradiates the sample in either an upright or inverted configuration.
In most patterning systems, a mechanical or piezo stage is used to move the sample during the laser irradiation. Such scanning stage systems cover wide areas to precisely move the samples in 3D, but the fabrication efficiency is limited by the scanning speed of stages. Another issue is residual vibration from movements. Alternatively, a dynamic mirror such as a galvano mirror can be used, which rapidly scans the laser beam across, while the scanning area is limited as a result of small angular changes. Galvano mirror scanning is popular in polymer fabrication because the high photosensitivity of these materials requires the avoidance of prolonged exposure to the beam. Combination of the scanning mirror and stage can be used for rapid volume fabrication.
As another alternative, a spatial light modulator (SLM) can be employed to control the light amplitude and phase to spatially shape the laser beam for multi-focal patterning or single-shot pattern projection
34
,
39
,
47
,
57
,
58
,
60
,
64
,
65
. Light modulation is achieved either by a digital mirror device (DMD) composed of an array of individually rotatable micro-mirrors, or a liquid crystal display (LCD) composed of an array of individual liquid crystal pixels electrically modulating the light. Light modulation is limited by the loss of light intensity resulting from interferences of individual lights modulated in amplitude or phase when shaping the light into the desired pattern. Single spot projection patterning with SLM is a maskless technology that allows flexible shaping of the amplitude in space and thus permits dynamic lithography. Direct writing is a slow process because the patterning is performed by scanning with a focused laser spot, while multi-focal patterning or pattern projection using SLM can enhance the throughput of laser direct writing.
Typically, a high numerical aperture (
NA
) objective lens, in particular a 100× water or oil immersion lens with
NA
=1.3–1.4, is used to focus the beam at the precursor material. In
Table 2
, feature sizes were obtained by using these high-
NA
objective lenses. In principle, the feature sizes of fabricated elements are determined by the spot size obtained by focusing with the objective lens. The full width at half maximum (FWHM) size of the focal spot (which represents the diffraction limit) in the lateral direction (plane perpendicular to the laser beam axis) is given by 1.22
λ
/
NA
, where
λ
is the laser wavelength. Employing the objective lens with
NA
=1.4, the focal spot size can be calculated to be ca. 700 nm for the wavelength of 800 nm. The feature sizes obtained using multi-photon cross-linking can be less than the focal spot size of the focused laser due to the Gaussian beam profile of the laser beam and the fabrication threshold for the process
31
, as shown schematically in
Fig. 1a
. Theoretically, the feature sizes can be decreased without no limitation by setting the laser intensity at the center of laser beam to the fabrication threshold as much as possible. However, in practice, around 200 nm is the minimum size due to pulse-to-pulse fluctuation of laser energy and instability of the scanning system as summarized in
Table 2
.
Figure 1b
shows that feature sizes in volume are determined by the laser writing scheme. In a free space, features of voxel becomes ellipsoidal shapes due to mismatch between focused spot sizes and Rayleigh lengths. On the substrate, surface bound structures are formed, as indicated by solid and dashed lines, depending on the position of focus. Determining resolution as the smallest distance between distinguishable structures, well-connected 3D structures can be formed by scanning the focused laser beam with a step smaller than the resolution.
Irradiation conditions
From
Table 2
, it is evident that various lasers have been employed for the cross-linking of proteins, including oscillator, mode-locked, Q-switched micro, solid state and fiber lasers. Q-switch lasers with picosecond pulses in the sub-nanosecond range are used for basic cross-linking, while femtosecond oscillators are utilized for microstructure fabrication. Longer pulse width causes heat generation and diffusion which induces irregularities such as spontaneous outbursts as well as thermal cross-linking, termed heat effect. Shorter pulse width is desirable because the heat effect can be minimized. Furthermore, most proteins have a denaturation temperature, at which their tertiary structure dissolves and function is lost. Therefore, shorter pulse width is preferred to ensure well-controlled fabrication quality and also prevent heat related protein denaturation. Both picosecond and femtosecond lasers create comparable feature sizes
45
. The femtosecond lasers available at present can be operated at considerably higher frequencies compared to picosecond lasers due to less heat accumulation effect, which increases the process speed. Picosecond lasers are affordable and compact, and thus also have applications in some fields. In addition, nanosecond lasers have been used for single shot cross-linking in conjunction with SLM-design holography
54
,
65
. The losses of light intensity resulting from complex interference patterns when using an SLM for 3D design requires can possibly be compensated for by heat associated with longer pulses, but it influences the cross- linking dynamics and quality of cross-linked proteins.
Near-infrared wavelengths are often elected because they are not absorbed by common photoinitiators such as rose bengal (RB) and methylene blue (MB), which is essential to inducing multi-photon absorption. Lasers with shorter pulse widths of approximately 100 fs are preferred in order to reduce any possible thermal effects, since both heat generation and cavitation bubbles in the aqueous environment can obstruct or degrade the fabrication process. When using mechanical or piezo stages, low laser energies in conjunction with high repetition rates with laser powers below 60 mW are adopted to reduce heat generation, while low scanning speeds on the order of μm/s are employed to allow sufficient time to induce cross-linking. Depending on the optional use of the galvano scanning or DMD projection techniques, different exposure doses or scanning speeds were chosen to adjust for the respective technique.
Solvents
The aqueous systems used in these processes typically are buffered solutions or commercially available protein stabilizing solutions. Organic solvents have also been reported, although these solvents could potentially modify the original tertiary structure of the protein and thereby its functionality could be impaired prior to fabrication. The addition of dimethyl sulfoxide (DMSO) has been shown to improve both viscosity and feature sizes
34
, although higher concentrations of DMSO in the system can also affect the tertiary structure or even denature the protein
66
. It is unclear why DMSO improves feature sizes and fabrication quality, although the heat and electron transfer properties of this solvent might play a role, in addition to the attendant increase in the viscosity of the precursor solution. In order to mimic these features, a glycerol-water solvent was employed in a prior work
37
. Because high concentrations of glycerol can be used to preserve proteins
71
, this solvent does not risk damaging the protein at any concentration. When fabricating in glass microfluidic channels, light refraction and aberration due to refractive index changes between device and protein precursor material caused an increase in feature sizes and limited penetration depth
37
. Use of glycerol-water solvents provided an improved refractive index match to glass with
n
=1.51 from pure water
n
=1.333 to over
n
=1.398, resulting in nearly unchanged feature sizes
37
. Additional investigations of refractive index matching and viscosity optimization in conjunction with proteins could allow glycerol-water solvents to be used to refine the fabrication process
72
.
Photoactivator systems
Photoactivator involves molecules of either photoinitiator or photosensitizer that upon light absorption undergo reaction to create a reactive species or a chemical change in another molecule, respectively. The reactive species or the chemical change is relevant in the subsequent light-independent processes, here cross-linking. The efficiency of photoactivator is defined as the ratio of activated molecules, here cross-linked molecules after initiation, to all available molecules. The higher efficiency allows faster scanning speeds applicable during fabrication. The efficiency depends on the light absorption properties as well as the number of reactive species generated by the photoactivator.
Classical biological dyes, including MB, RB, rhodamine and eosin-y, are typically used to demonstrate fabrication principles and to understand the cross-linking mechanism. These dyes are known for their good absorption properties and their ability to generate radicals. Conjugated dyes such as Texas Red and fluorescein isothiocyanate (FITC) have high absorption coefficients and also enhance cross-linking.
There have been on-going attempts to identify efficient photoinitiators to allow the industrialization of polymer cross-linking and 3D printing. The use of effective photoinitiators could improve the fabrication process and also expand the range of applicable proteins. As an example, a benzophenone derivative (BPD) was used to cross-link small amounts of various types of collagen
52
, while the water-soluble compound sodium 4-[2-(4-morpholino)benzoyl-2-dimethylamino]butylbenzenesulfonate (MBS) was utilized to cross-link enhanced green fluorescent protein (EGFP)
37
. A three-component system comprising a protein, rhodamine B and flavin adenine dinucleotide (FAD) significantly improved both fabrication speed and feature sizes
66
. However, additional increases in efficiency could increase the risk of generating radicals that would lead to cytotoxicity during the fabrication, as well as chemical leaching that is undesirable in various applications.
Due to their long triplet state lifetimes, with moderate radical generation, FAD and flavin mononucleotide (FMN) have been used for
in situ
cell applications over short time spans
56
. Several studies involving low photoinitiator concentrations have demonstrated that the threshold protein concentration required for fabrication increases dramatically
32
,
33
,
68
. Fabrication without photoinitiators has also been reported
32
,
33
,
49
,
68
, although some attempts were unsuccessful
48
. Such discrepancies in prior works indicate that controlling all the major factors such as laser properties, irradiation conditions, solvent, photoactivator and protein concentration that can affect the outcome of the process is the key to achieving repeatability.
Proteins
High concentrations of protein precursor materials (which are discussed in more detail in Section: Structures and functions of cross-linked proteins) are required for cross-linking. These high concentrations are best achieved by dispersing the required amount of protein powder in a small solvent volume, following by stirring or shaking for a prolonged time period to ensure complete mixing. Even at elevated concentrations of 400 or 600 mg/mL in conjunction with a relatively large amount of a photoinitiator, fabrication appears to be possible only when applying a slower scanning speed relative to that used during polymer cross-linking. Low concentrations of proteins still allow for cross-linking but increase the fabrication threshold and require the use of higher photoinitiator concentrations
33
. Phragma dialysis can increase protein concentrations, but can only be applied for several repetitions due to the physical take-off volume limit. An alternative demonstrated by several groups involves mixing bovine serum albumin (BSA) with other proteins to retain the function of these other proteins while inducing cross-linking
32
,
38
,
43
,
56
.
The protein concentration also significantly affects various irradiation parameters, in particular the penetration depth of the laser beam. Protein solutions may appear slightly colored, but are still transparent. Higher protein concentrations inevitably lead to greater beam absorption and scattering at shallower penetration depths. Cross-linking at deeper regions can be accomplished by controlling the laser light dose applied, although the protein cross-linking is quickly saturated at the surface. In addition, the aqueous protein solutions described above can be sensitive to the heat generated at higher laser powers. Unfortunately, the already low scanning velocities cannot be further reduced while retaining practical fabrication processes.
Proteinaceous microstructures have been found to have relatively low Young's modulus values in the range of 0.5–4 MPa
43
,
45
,
63
, leading to poor mechanical stability that makes it difficult handle and preserve these structures. There are two established means of obtaining protein-polymer hybrid structures that combine the mechanical strength and chemical inertness of the polymer with the functionality of the protein. One approach is to conjugate the protein with acrylate, allowing the polymer chemistry to govern the cross-linking process
44
. This procedure has been successful employed with high molecular weight cellular matrix proteins such as collagen. The other approach uses protein-polymer adhesion after sufficient roughening of the polymer surface, such that the native protein does not undergo chemical changes
43
.
Structures and functions of cross-linked proteins
In this section, the protein properties that are necessary for cross-linking are discussed based on representative proteins chosen from the protein data bank (PDB)
73
, as indicated in
Table 1
. The role of BSA as a standard material and the function retention of cross-linked proteins are also addressed.
PDB
When considering cross-linking, it is important to understand where and how the cross-linking takes place, as this enables us to chemically control the microstructure growth of modified or artificial proteins.
Figure 2
shows the secondary structures, unique chains, chain lengths, total unit weights and amino acid counts within the protein sequences of 16 different protein structures, all obtained from selected PDB data files. These properties reflect protein tertiary structure, stereo-accessibility, and potential sensitivity for cross-linking processes.
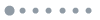
Figure 2.PDB data overview.Properties of 16 different protein structures obtained from selected PDB files: (a) the secondary structures, (b) unique chains, (c) chain lengths, (d) unit weights, and (e)–(f) amino acid distributions. (e) Single letter amino acid codes sorted by classification according to Livingstone et al. from ref. 74. (f) The distributions of amino acid occurrence in the PDB FASTA sequence for 16 proteins versus respective database values, calculated from reports by Eitner et al. from ref. 75.
Secondary structure features such as alpha-helices, beta-sheets and unassigned parts are associated with certain groups of amino acids, but also give rise to stereo-accessibility or lack thereof.
Figure 2a
shows that there is no clear trend amongst these proteins. In order to connect ordered secondary structures, a protein always has some unassigned, likely unstructured regions and so it is not surprising to find approximately 50% unassigned chains. Among this small selection of proteins, the two proteins 3HQV and 3GHG have considerably more unassigned sequence parts while proteins 4F5S, 2CMM and 1OCC have relatively few unassigned sequence parts. There also seems to be no trend regarding alpha-helices and beta-sheets that would be beneficial for cross-linking.
The number of unique chains, so-called subunits, is depicted in
Fig. 2b
. This value indicates how many chains may not be covalently bound, although other forces may lead to interactions between the chains that constitute the proteins used to acquire the PDB data. It is evident that most have one unique chain. This trend is expected since non-covalently bound protein chains might not be fully included in the cross-linking and thus tend to render the tertiary structure of the protein incomplete and disrupted. Interestingly, there is also one extreme example of successful protein cross-linking with cytochrome C, where 13 unique majorly helical chains interact to form the protein. It would be challenging to cross-link proteins that have associated metal units, but the 13 unique chains of cytochrome C suggest that even fabrication of complex proteins might succeed. In fact, Connell et al. reported the successful cross-linking of myoglobin, which binds iron via porphine in a similar manner to the red blood cell protein hemoglobin
47
. It is unclear whether appropriate iron was available during the fabrication or if iron porphine was associated throughout the process in the prior work, but it is likely that supplying the appropriate quantity of iron porphine after fabrication would lead to reconstituting iron complexes in the proteinaceous microstructure.
Protein chain lengths and weights are summarized in
Figs. 2c
and
2d
, which also show no clear trend in terms of length, weight or the ratio of these two factors. It was previously assumed that, in general, molecular weight affects cross-linking and higher weights should result in a lower fabrication threshold. Pitts et al. provided results comparing fibrinogen with BSA
32
and showed that, although the molecular weight of fibrinogen is much higher than that of BSA, only at low photoinitiator concentration fibrinogen cross-linking occurred 2–10 times faster. This observation demonstrates that molecular weight is not necessarily an important factor. It is probable that stereo-accessibility and amino acid presentation are more important to successful cross-linking.
Figures 2e
and
2f
can be used to determine differences in the amino acid distributions of the 16 different proteins, based on the amino acid counts reported by Eitner et al.
75
In order to reasonably summarize the amino acids from individual counts, we followed the amino acid classification suggested by Livingstone et al.
74
The classifications and respective counts of amino acids are shown as single letter codes in
Fig. 2e
. These same single letter codes are also used to describe the FASTA protein sequences in the PDB. Counting the single letter codes within all sequences results in the distributions represented by the box plot graphs in
Fig. 2f
. The amino acid counts within the entire database were obtained from ref.
75
and are summarized by the same classifications. The results demonstrate that the proline distribution is quite similar to the database value. It should be noted that the proline content of Col. Ⅰ is significantly different from the median and contributes to the broadening of the distribution. Possibly, with more reports of cross-linked proteins, the distribution might align fully with that of proline. In addition, all aromatic amino acids, aliphatic amino acids, amino acids with accessible sulfur residues (with S (serin) excluded), and positively and negatively charged amino acids score higher than the database values by about a quartile of their distributions. Again, this result could still change with more cross-linked protein entries. It is, however, interesting to note that amino acids with residues that could undergo radical or ion chain reactions score higher. This higher availability might hint at the protein cross-linking mechanism(s). Finally, so-called tiny amino acids that are not expected to contribute to any potential cross-linking mechanism show significantly higher counts in the general database and even lie outside of the distribution of the cross-linked proteins. Scanning the protein sequence for notably higher amino acid counts disregards the importance of stereo-accessibility, but could provide some initial insights into relevant amino acids.
Retention of protein function
One or several functions of a protein are generated by an active functional domain in the protein structure. As far as currently understood, the cross-linking process is random, it can affect the functional domain of a protein such that the individual protein molecules lose their function. However, written submicron-sized lines of protein would consist of many individual protein molecules which could still retain some function on a statistical basis. Therefore, the retention of function also depends on the type of protein and robustness in response to changes in or near the functional domains.
Figure 3
illustrates a fictive example comparing laser induced cross-linking and surface adhesion for biological applications of cell adhesion
43
. When a protein adheres to a surface, depending on the surface charges and potentials, the protein can lose function due to structural deformation or simply because the functional domains are rendered inaccessible.
Figure 3a
shows a protein with a functional domain, and
Fig. 3b
illustrates the manner in which potential conformational changes affect the function retention of that domain. Understanding that a conformational change can either significantly inhibit functioning or still allow full activity, direct laser writing processes are compared with surface adhesion in
Figs. 3c
and
3d
. Direct laser writing is assumed to be a randomized process in which some molecules undergo large conformational changes while others undergo only minimal changes. Using cell adhesion to the protein as an example, the grey arrows demonstrate that some parts of the direct laser write-fabricated element repel the cells because the protein function is impaired, while other areas support cell adhesion because function is retained. In the case of the surface adhesion of the protein to four different surfaces, the surface charge and potential lead to more highly organized structures in which the functionality is either completely retained (surface 1) or completely impaired (surfaces 2–4), depending on the extent to which the surface potential of the protein leads to adhesion. This figure demonstrates the manner in which the randomness of the cross-linking process makes the fabricated element more likely to retain the protein function.
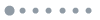
Figure 3.Proposed processes for protein cross-linking and adherence.A schematic illustration depicting the differing degrees of deformation and adhesion that can degenerate protein functionality. Specific surfaces render the functional domain inaccessible or can have little effect on the native protein, leading to retained protein function. Figure reprinted with permission from supplementary material of ref. 43, copyright 2017, American Chemical Society.
There have been various reports documenting the retention of binding function. Kaehr et al. showed that binding fluorescence is proportional to the protein concentration
56
, implying not only retained function but also a non-preferential process. There have also been some reports about the retention of protein enzymatic functions
35
,
36
,
38
. However, to date there have been no analyses of protein activity or quantitative assessments of active units. It would be challenging to actually perform such experimentation, since the surface area and availability of the protein can affect the results, thus differences between bulk and laser-fabricated samples could be important.
Biodegradation of these materials has also been unexplored. Assuming that the cross-linking proceeds as a random process, one would expect that the resulting structures would be degradable because the majority of normal peptide or organic bonds would be retained. Sun et al. showed changes in proteinaceous structures within two weeks of exposure to collected raindrops, such that the surface became rougher, small features were reduced and structural elements seemed less supportive
41
. These observations suggested that complete degradation could occur within several months, although this was not confirmed.
Bovine serum albumin as a common standard
Table 1
confirms that almost all prior studies utilized bovine serum albumin (BSA).
Figure 2
demonstrates that BSA (4F5S) consists primarily of alpha-helix components and that the molecular weight and height of this material match the common average values of the current protein group. Additionally, BSA is an affordable, abundant blood serum protein, and is a good representative of the average properties of such materials
76
.
BSA also exhibits several other interesting properties as a blood regulating protein. Because BSA is found in blood, in which clotting is undesirable, BSA does not show specific binding preferences, although it will undergo non-specific binding to surfaces such as other proteins. Harper et al. noted that BSA was also selected in their work as a protein monomer due to its oxygen radical scavenger functionality
57
.
Because of its availability and previous reports, BSA fabrication parameters have been well studied. It allows to become a standard to introduce and discuss variation of the major factors including laser properties, irradiation conditions, solvent, photoactivator and protein concentration, that can affect the outcome of the process. BSA is also often used due to its unique properties, one of which is pH actuation capability
39
,
40
,
62
, as discussed later. Kaehr et al. have also shown its potential to be mixed with other proteins and create a binding gradient that reflects the retained function of other proteins
39
.
Cross-linking mechanisms
The cross-linking mechanisms associated with these techniques are not yet fully understood, although we can discuss various key aspects of these mechanisms. In the field of polymer science, multiple-step photo-induced reaction mechanisms are readily available to explain the growth dynamics of cross-linking. In the case of acidic pathways, organic monomers become acidic upon photo-excitation and interact with an aqueous base developer, while during hydrosilylation platinum colloids act as a catalyst after UV decomposition. In the case of radical chain reactions, radicals are generated by light irradiation and subsequently promote cross-linking while being regenerated.
Radical chain reactions involve unique dynamics due to the propagation and termination phases, which differ from both the acidic and catalytic mechanisms. Radical mechanisms tend to exhibit increased fabrication rates and can provide specific polymerization morphologies. This technique is commonly used for polymer cross-linking and has also been investigated as a possible mechanism for protein cross-linking. As a side note, one should keep in mind that the cross-linking of specific proteins with varying tertiary structural features could follow other mechanisms, including acidic mechanisms. These alternate mechanisms could involve acidic amino acids such as glutamate and aspartate or catalytic mechanisms based on metals such as iron or manganese as cofactors associated with the protein complex.
A radical chain reaction consists of three steps. The first is initiation, in which radicals are generated via irradiation. Propagation follows, during which radicals are generated simultaneous with growth of the polymer via interactions between radicals and monomers. Finally, termination occurs when radicals react with one another or with other compounds. Free radical initiated cross-linking reactions are typically exothermic
79
.
Applications of proteinaceous microstructures
Due to the vast number of native and artificially engineered proteins, the potential for applications seems limitless. Current applications depend on either pH-actuation and soft optics, in which proteins are manipulated as deformable materials, or cell culture and microfluidics, where protein functions are harnessed. Enzymatic reactors have been developed to demonstrate this concept, and by choosing an applicable enzyme microfluidic enzymatic reactors might become important. Although no drug delivery applications have yet been reported, binding affinities could be utilized for enhanced recognition, as required in targeted delivery, or reduced recognition, such to reduce immune responses. In the near-future the retained functions of proteins could contribute to disease treatment or to the bottom-up rebuild of structures impacted by disease.
Conclusion
The fabrication of 3D proteinaceous microstructures is an advancing field with great potential, due to the consequent retention of protein function and the availability of a vast range of different types of proteins. Similar to the field of polymer science, protein cross-linking is increasingly growing as a research subject. The associated mechanisms and the key protein properties that allow convenient fabrication have not yet been identified. However, even without this systematic understanding, some proteins have been utilized to execute specific applications.
The applications reported herein can be divided into two categories. One takes advantage of the deformability of proteins to obtain pH-activated devices and soft optics. The other uses binding affinities or enzymatic reactivities for cell culture and microfluidic integration. Furthermore, because binding affinity might induce swelling in response to molecules similar to the reported response to pH changes, a combination of both principles seems feasible. Protein cross-linking may also be a useful means of capturing live cells
in situ
. Combining protein functions with specific structural designs is expected to greatly expand the applications of this technique.
Acknowledgements
This work was partially supported by the RIKEN SPDR program. This work was partially supported by The Amada Foundation Research Grant (Jyuten-Kenkyu-Kaihatsu-Josei A).
Competing interests
The authors declare no competing financial interests.