1 Introduction
Low profile and vertically polarized phased array antennas with end-fire radiation patterns are highly demanded for modern airborne communication and radar applications. Because of the large wavelength in the ultra-high frequency (UHF) band, traditional vertically polarized antennas in the UHF band usually have relatively large profile height. Evidently, antennas with large profile height will destroy the aerodynamic and electromagnetic stealth performance of airborne platforms [1].
In recent years, many approaches have been developed to reduce the profile height of vertically polarized end-fire antennas. In Ref. [2], a novel end-fire antenna mounted on a large metallic surface was presented. The end-fire antenna in Ref. [2] consists of a trapezoidal launcher and six rows of coupled microstrip resonators with different lengths. It possesses low-profile height of 0.044λl and 109% bandwidth, where λl is the wavelength at the lowest frequency. However, in this design, the space between the radiating aperture and the ground plane should be filled with thick dielectric materials, which prohibits its operation in lower frequencies like the UHF band. A microstrip Yagi-Uda antenna array with end-fire radiations and vertical polarization was developed in Ref. [3]. The Yagi-Uda antenna consists of magnetic dipoles that feature with very low profile height (~0.027λl). However, it only has a limited bandwidth of 13.1% for the voltage standing wave ratio (VSWR) less than 2.0. The substrate integrated waveguide (SIW) H-plane horn is another candidate for low profile and vertically polarized antenna designs [4,5]. An innovative H-plane horn antenna based on the SIW horn was presented in Ref. [5]. It achieves low profile height of 0.09λl and an 8.11% relative bandwidth for VSWR < 2.0. Obviously, the limitation of this design is also the narrow bandwidth. Surface-wave antennas are promising for wideband end-fire antenna designs [6,7]. In Ref. [6], a flush-mounted surface-wave antenna with 120% impedance bandwidth was presented. However, the antenna has vertical height of 0.127λl, which is relatively large and may be difficult to mount over airborne platforms for conformal designs. Another surface-wave antenna with a grounded ceramic slab (εr = 25) and a wideband surface-wave launcher was developed in Ref. [7]. The profile height is 0.065λl and the impedance bandwidth is around 98.7% for VSWR < 2.0. However, ceramic slabs with large sizes are usually not available in the market and always lead to heavy weight.
As can be observed, requirements on low-profile, wide bandwidth, and vertical polarization impose many challenges on end-fire antenna and its array developments in the UHF band. As a type of frequency-independent wideband antenna with end-fire radiations, log-periodic dipole antennas have attracted much interest in the antenna community [8,9]. Its profile height mainly comes from the metallic cylinders rather than dielectric slabs as mentioned earlier. The height of the conventional monopole antenna is around λ/4, where λ is the free-space wavelength at the operating frequency [10]. There are various efficient approaches developed for profile height reduction, including top-hat loading [11], meandering the arms of monopoles [12], fractal designs [13], employing the AMC structure [14,15], etc. The metallic cylinders are much lighter in weight than the dielectric slabs and are more attractive to implement in airborne communication systems. Log-periodic monopole arrays are thus suitable to install over metallic platforms for vertically polarized and end-fire radiations in the UHF band.
On the other hand, in some particular cases, antennas are not allowed to protrude out of airframes to keep aerodynamic and electromagnetic stealth performance. Integration of antenna arrays into a cavity over a metallic platform is required. This strategy avoids destroying the original airframe of an aircraft. However, phased arrays with vertically polarized end-fire scanning beams that are suitable to embed in cavities are rarely reported in the literature.
In this paper, a low profile, wideband, cavity-embedded antenna array with vertically polarized end-fire scanning beams operating at the UHF band is developed. The array consists of 16 log-periodic top-hat monopole array antenna elements with low-profile height of 0.062λl. The width of the array antenna element is less than 0.185λl, thus, it is compact enough to eliminate grating lobes in linear scanning arrays. The performance of the array is also investigated when it is embedded within a cavity for the sight-invisible integrated installation on metallic platforms. The simulations demonstrate that the cavity-embedded uniformly spaced linear array (1×16) is able to scan up to ±45° in the horizontal plane and operate from 300 MHz to 900 MHz for VSWR < 2.0. The prototype of the proposed log-periodic top-hat monopole array antenna element and its uniformly spaced linear array (1×2) were fabricated and validated experimentally. The measured results agree well with the simulation results.
2 Design principle and antenna configurations
2.1 Log-periodic top-hat monopole array antenna element and array
Fig. 1 (a) shows the geometry of the end-fire antenna element. In the initial design step of this log-periodic monopole array, the spacing factor and the scale factor of the monopoles in the proposed element are chosen as σ = 0.89 and τ = 0.08 following the procedures in Ref. [16], respectively. These two design parameters will be optimized in further steps to realize wide impedance matching and end-fire radiation patterns. Two types of monopole elements are employed, including non-loaded monopoles operating at the higher band, and top-hats-loaded monopoles operating at the middle band and lower band. For the non-loaded monopoles operating at the higher frequency, the height of monopoles can be estimated as 1/4 wavelength corresponding to its resonant frequency, while the top-hat-loaded monopoles introduce capacitance to keep the same height with the non-loaded monopoles and operate at the middle band and lower band according to Ref. [16]. The top-hat loadings introduce capacitive loading to the monopole antennas and effectively decrease the lowest operating frequency of monopoles [16–18]. In other words, the top-hat loadings reduce the profile height of the monopole antennas for a given resonant frequency. As can be seen from Fig. 1 (a), the proposed antenna element is composed of a coupled feeding structure, a co-planar paralleled strip line, top-hat loaded monopoles, and two 100 Ω lumped resisters at the end of the feeding line. Three types of dielectric substrates are employed in the design. Specifically, the dielectric substrate 1 (εr1 = 2.2, tanδ1 = 0.0009, and t1 = 10.00 mm) is utilized to support the 12 top-hat loaded monopoles. In addition, the co-planar paralleled strip lines are etched on the top surface of the dielectric substrate 1 while a finite ground plane is printed on the bottom surface. The dielectric substrate 2 (εr2 = 10.2, tanδ2 = 0.0035, and t2 = 3.81 mm) is utilized to fabricate the coupling feeding strip lines for exciting the differential feeding lines. The top-hat loadings for the monopoles are metallic patches and are printed on dielectric substrate 3 (εr3 = 2.2, tanδ3 = 0.0009, and t3 = 0.51 mm).
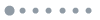
Figure 1.Configuration of the proposed log-periodic top-hat monopole array antenna: (a) perspective view of the array antenna element; (b) side view of the array antenna element; (c) perspective view of the proposed antenna array without cavity embedding.
The proposed log-periodic monopole array antenna element is composed of 12 vertically polarized, top-hat loaded monopoles with an identical low-profile height of 52.00 mm (~0.052λl). Each monopole operats at different resonance frequencies from the highest (about 900 MHz) to the lowest (about 300 MHz). As it can be observed from the inset in the top left corner of Fig. 1 (a), the top-hat loading is employed to reduce the height of the monopoles, and the configration of rectangle-shape top-hats is carefully designed to minimize the transverse dimension, and meanwhile avoid overlapping between adjacent monopoles while keeping the resonant frequency unchanged. All of the top-hat monopoles are formed by metallic cylinders with the same diameter dp and profile height hp. The resonant frequencies of the ith monopole and the (i–1)th monopole are related by the formula fi = τfi–1, where fi and fi−1 are the resonant frequencies of the ith and (i–1)th monopoles, respectively [10,18,19]. Particularly, in order to enhance the front-to-back ratio in radiation patterns, the first two monopoles (i = 1, 2) are designed to operate at the same resonant frequency (operating around 900 MHz), and the last two monopoles (i = 11, 12) are also designed to operate at the same resonant frequency (operating around 300 MHz).
As illustrated in the bottom right inset in Fig. 1 (a), a feeding structure formed by coupling strip lines is designed to produce differential phase excitations for adjacent monopole antennas. The guided waves supported by the coupling strip lines are excited by coaxial cables and are coupled to the co-planar paralleled strip lines by a cross-overlapped section. A quasi-differential phase excitation is thus achieved between the co-planar paralleled strip lines. The cross-overlapped section consists of a curved strip line with a sector shape and the co-planar paralleled strip lines. The gap distance g between the co-planar paralleled strip lines plays an important role in bandwidth enhancement and impedance matching. Good impedance matching can be obtained by adjusting the width of the co-planar paralleled strip lines. Like the design in Ref. [16], capacitance could move the resonant frequency to a lower frequency band. The introduction of greater capacitance results in a decrease in impedance, leading to an increase in the width of the microstrip line for impedance match. Thus, zigzag-shaped co-planar paralleled strip lines manifest as a consequence. Two 100 Ω lumped resistors are introduced at the end of each co-planar paralleled strip line to absorb the redundant energy.
Fig. 1 (b) and Fig. 1 (c) show the side view and the perspective view of the array without cavity embedding, respectively. It is worth mentioning that the width of the proposed log-periodic monopole array antenna element is elaborately optimized to guarantee grating-lobe-free scanning as well as to avoid structure overlapping between adjacent elements. Rectangle shape as top-hats was chosen, rather than other shapes, to introduce more capacitance compared with other shapes of top-hats with the same transverse dimension. The length and width of the rectangle shape top-hats are elaborately designed. Particularly, expanding the length of the rectangle shape preferentially, rather than the width more capacitance needs to be introduced. Unified shapes of top-hats with limited small transverse dimensions are guaranteed in this design. Fig. 1 (c) shows the perspective view of the proposed antenna array without cavity embedding. As the antenna elements in the proposed 1×16 uniformly spaced linear array are arrayed along the X-axis, the end-fire radiation is in the direction of the –Y-axis (0 in horizontal plane). The edge-to-edge distance between the adjacent elements is 0 while the distance between the adjacent coaxial cable excitation ports is Wb = 185.00 mm.
2.2 Cavity embedded antenna array
Although the profile height of the antenna array has been reduced a lot as compared with designs in Refs. [20–22], the physical height (hp + t1 = 62.00 mm) in low frequency bands like the UHF band is still not acceptable in many practical situations. For instance, antennas are not allowed to protrude out of airframes in systems demanding electromagnetic stealth and keeping good aerodynamic performance. Therefore, integration of antennas into a cavity over the airborne platforms is an alternative approach for such kinds of applications. Fig. 2 shows the configuration of the proposed 1×16 linear phased array embedded in a shallow cavity. As can be seen, the width Wc and length Lc of the cavity are a little bit larger than the transverse dimensions of the antenna array. The depth of the cavity hc is determined to ensure the top-hat loadings of the antenna array are conformal with the metallic platform it sits. The distance dc in front of the array is optimized to guarantee the energy is capable of propagating out of the cavity. The dimensions of the cavity are as follows: Lc =2000.00 mm, Wc = 3490.00 mm, hc = 62.00 mm, tc = 2.00 mm, dc = 440.00 mm, ds = 273.00 mm, and de = 272.00 mm.
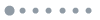
Figure 2.Configuration of the proposed 1×16 linear array within a cavity.
3 Performance of the proposed wideband low-profile antenna array
This section presents the simulation results of the proposed cavity-embedded scanning array, while the simulation results of the array antenna element will be presented together with the measurement results in Section 4 for concise and comparison purposes. The performance of the proposed wideband low-profile antenna array is simulated by the commercial electromagnetic simulation software Ansys HFSS 17.1.
Fig. 3 shows the active VSWR of the antenna element in an array environment and the peak realized gain of the 1×16 scanning linear phased array. As can be observed, the proposed antenna array is operated from 250 MHz to 900 MHz for active VSWR < 2.0. When the array scans up to ±45°, the active VSWR slightly varies but is still less than 2.0 over the entire operating frequency band. The simulated peak realized gain varies from 14.0 dBi to 20.4 dBi across the frequency band of 300 MHz−900 MHz when scanning from 0° to 45°. The peak realized gain drops rapidly at frequencies below 300 MHz. This realized gain drop is caused by the two lumped resistors at the end of the co-planar paralleled strip lines, as energies below 300 MHz are severely absorbed by the two resistors. The last several monopole posts resonating around 300 MHz are placed close to the two absorbing resistors. The energies, supposed to spread into the radiation space through the monopole posts in the end position, are partially absorbed by the resisters.
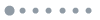
Figure 3.Simulated peak realized gain and active VSWR of the array.
Fig. 4 shows the simulated normalized radiation patterns in the XOY and YOZ planes at three typical frequencies (500 MHz, 700 MHz, and 900 MHz), respectively. It can be seen that end-fire radiation patterns are obtained. In the YOZ plane, the beams tilt about 30° from the XOY plane due to the electromagnetic reflection from the finite ground plane [2]. In the XOY plane, the front-to-back ratio is larger than 20.0 dB at the three typical frequencies. This design has a good performance in co-polarization natively, owning to the current is mainly distributed in the vertical direction along the monopoles as stated in Ref. [18]. The simulated cross-polarization components are 20.0 dB lower than their co-polarization counterparts.
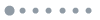
Figure 4.Radiation patterns of the proposed scanning array in (a) XOY plane and (b) YOZ plane.
Fig. 5 shows the electric field distributions in front of the proposed wideband low-profile log-periodic monopole scanning array at the center frequency of 600 MHz in the cases with and without cavity embedding. As it can be observed from Fig. 5 (a), there is no evident difference between the electric field distributions by embedding the antenna array in a cavity. In both of the two cases, the electric fields mainly travel toward the end-fire direction with nearly the same elevation angle. It can be also found that electromagnetic waves are capable of propagating through the walls of the cavity when the antenna array is embedded into a cavity. Therefore, the beam tilting in the YOZ plane is due to the electromagnetic reflection from the finite ground plane (the same phenomenon also occurred in Refs. [2,3,5,7,11], designs with vertical polarization end-fire beams) rather than the walls of the cavity. Fig. 6 shows the scanning performance of the proposed array in horizontal planes (XOY plane). It can be seen that the proposed array exhibits a wide scanning angle of ±45° at 300 MHz, 500 MHz, 700 MHz, and 900 MHz. Grating lobes are eliminated across the frequency band due to the optimized narrow width of the antenna array element.
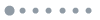
Figure 5.Electric field distributions in front of the array at the center operating frequency 600 MHz: (a) embedded into a cavity and (b) without cavity embedding.
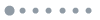
Figure 6.The scanning performance of the proposed array in horizontal planes: (a) 300 MHz, (b) 500 MHz, (c) 700 MHz, and (d) 900 MHz.
In summary, the simulation results demonstrate that the proposed end-fire antenna array achieves wideband and wide scanning ranges in the UHF band. Its low-profile and conformal characteristics make the designs a promising candidate for airborne/shipborne end-fire antenna designs applied in horizontal scanning and detection.
4 Experimental validations
It is hard to process and manufacture due to the large physical size of a 1×16 antenna array (2000.00 mm × 3490.00 mm). A prototype of a 1×2 linear array as shown in Fig. 7 was fabricated to experimentally demonstrate the wideband, low-profile, and cavity-embedded characteristics of the proposed antenna array. As can be seen from Fig. 7 (b), the profile height of the end-fire antenna element is 62.00 mm (including 10.00 mm thickness for dielectric substrate 1). Therefore, as can be seen from the measurement setup as shown in Fig. 7 (c), a shallow cavity with thickness tc = 2.00 mm, hc = 64.00 mm, Lc = 2000.00 mm, and Wc = 750.00 mm is fabricated for antenna array flush mounting.
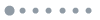
Figure 7.Prototype and measurement of the proposed end-fire antenna element and cavity-embedded array: (a) top view and (b) side view of the end-fire antenna element; (c) radiation pattern measurement setup for the cavity-embedded 1×2 linear array.
4.1 Measurement of the array antenna element
The simulation and measurement VSWRs of the proposed wideband low-profile end-fire array antenna elements are shown in Fig. 8. It can be seen that the simulated VSWR is less than 2.0 in an operating frequency band of 300 MHz−900 MHz. Both the measured VSWRs of the two fabricated end-fire antenna elements agree well with the simulated VSWR. The very slight difference is caused by the inevitable errors introduced in the fabrication and assembling.
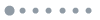
Figure 8.Simulated and measured VSWRs of the proposed end-fire array element.
The simulated and measured realized gain of the proposed wideband low profile, end-fire array antenna element is shown in Fig. 9. As can be observed, the measured peak realized gain at some particular frequencies is 1 dB lower than the simulated gain. The simulated radiation efficiency is larger than 60%. In addition to the errors introduced in the fabrication, assembling, and far-field measurement procedure, another important factor that contributes to the measured gain is the unknown loss tangent of the FR4 dielectric slab in the UHF band. Moreover, the standard frequency range of the chamber in our institution is applicable to the frequency band of 700 MHz–40 GHz. It also partially contributes to the measurement errors for frequencies below 700 MHz.
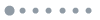
Figure 9.Realized gain and radiation efficiency of the end-fire array element.
The normalized radiation patterns of the proposed antenna element at three typical frequencies are shown in Figs. 10 (a)–(f), respectively. The measured and simulated co-polarization radiation patterns agree well with each other in both the XOY and YOZ planes. The radiation patterns in the XOY plane show that the main beams radiate toward the end-fire direction (−Y-axis), while there is a 30° beam tilting in the YOZ plane due to the ground plane. This beam tilting phenomenon is similar to the other end-fire antennas [3,18]. Moreover, it can be also found that the proposed antenna element offers a front-to-back ratio (FTBR) of 20 dB at 500 MHz. FTBR is larger than 25 dB and 15 dB at 700 MHz and 900 MHz, respectively. In the end-fire direction, the cross-polarization component is 20 dB lower than its co-polarization counterparts.
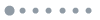
Figure 10.Simulated and measured normalized radiation patterns of the proposed array element: (a) XOY plane@500 MHz, (b) YOZ plane@500 MHz, (c) XOY plane@700 MHz, (d) YOZ plane@700 MHz, (e) XOY plane@900 MHz, and (f) YOZ plane@900 MHz.
4.2 Measured results of the proposed cavity-embedded 1×2 linear array
As shown in Fig. 7 (c), the two end-fire antenna elements are arrayed along the X-axis and embedded into a shallow cavity for measurement.
The active VSWR of the proposed 1×2 linear array is presented in Fig. 11. The simulated and measured active VSWRs of the linear array again indicate that the proposed array possesses a wide frequency band across 300 MHz−900 MHz with active VSWR < 2.0. The simulated and measured realized gain of the proposed cavity-embedded 1×2 linear array is shown in Fig. 12. As it can be seen, the simulated gain ranges from 8 dBi to 11 dBi, while the measured gain at discrete frequency points ranges from 5.8 dBi to 10.0 dBi. The simulated radiation efficiency is larger than 60% in the entire frequency band and illustrates an average radiation efficiency of 80% across the frequency band of 400 MHz to 900 MHz. There exists some slight difference because of the inevitable errors introduced in the manufacturing and assembling. However, the agreement between the simulation results and experimental results is acceptable.
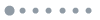
Figure 11.Simulated and measured VSWRs of the cavity-embedded 1×2 linear array.
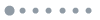
Figure 12.Realized gain and radiation efficiency of the cavity-embedded 1×2 linear array.
The radiation patterns of the proposed array were measured. Figs. 13 (a)–(h) compares the measured and simulated radiation patterns at 300 MHz, 500 MHz, 700 MHz, and 900 MHz, respectively. The measured and simulated co-polarization radiation patterns agree well with each other in both the XOY and YOZ planes. The radiation patterns in the XOY plane show that the main beams radiate toward the end-fire direction (−Y-axis), while the radiation patterns in the YOZ plane tilt about 30° from the ground plane. The proposed array offers FTBR larger than 20.0 dB and 10.0 dB at 500 MHz and 900 MHz, respectively. Again, in the end-fire direction, the cross-polarization component is 20 dB lower than its co-polarization counterparts. The measured radiation patterns again demonstrate the vertically polarized end-fire radiations of the proposed cavity-embedded antenna array.
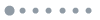
Figure 13.Simulated and measured normalized radiation patterns of the proposed array: (a) XOY plane@300 MHz, (b) YOZ plane@300 MHz, (c) XOY plane@500 MHz, (d) YOZ plane@500 MHz, (e) XOY plane@700 MHz, (f) YOZ plane@700 MHz, (g) XOY plane@900 MHz, and (h) YOZ plane@900 MHz.
Table 1 compares the performance of the proposed low profile, wideband log-periodic top-hat monopole antenna array with other conventional vertically polarized end-fire antennas. As can be observed from Table 1, the shortcoming of the low-profile end-fire antennas using coupled microstrip resonators in Ref. [2] is its large transverse dimensions (0.752λl). Similarly, the end-fire antennas in Refs. [3,18] exhibit low profile height but have relatively large widths 0.98λl and 0.92λl, respectively. These large transverse dimensions limit their applications in phased antenna array development for wide/moderate scanning without grating lobes. In contrast, the vertically polarized log-periodic top-hat loaded monopole antenna array in this work achieves end-fire radiation over the wide operating frequency band of 300 MHz−900 MHz. The bandwidth is comparable to the designs in Ref. [2], but our work features compact configurations.

Table 1. Performance comparison of end-fire antennas with vertical polarization radiation patterns.
Table 1. Performance comparison of end-fire antennas with vertical polarization radiation patterns.
| Transverse dimension (λl) | Profile height (λl) | Bandwidth | Available for scanning array development? | *The profile height in this work can be regarded as zero in cavity embedding cases. | [2] | 0.752 | 0.044 | 109.0% for VSWR<2.0 | × | [3] | 0.98 | 0.025 | 13.1% for VSWR<2.0 | × | [4] | 0.235 | 0.152 | 76% for VSWR<2.5 | × | [5] | 1.209 | 0.09 | 8.1% for VSWR<2.0 | × | [18] | 0.92 | 0.036 | 20.5% for VSWR<2.0 | × | This work | 0.185 | 0.062* | 100.0% for VSWR<2.0 | √ |
|
On the other hand, if we attempted to scale the designs in Refs. [2−5] to the UHF band (for example 300 MHz−900 MHz), it would result in bulky and heavy antenna structures as thick dielectric substrates are required to fill up the spacings, the height dielectric substrates of design in Ref. [2] would be 45.00 mm, 25.49 mm for design in Ref. [3], 152.00 mm for design in Ref. [4], and 71.63 mm for design in Ref. [5], respectively, while only 10.00 mm thickness for dielectric substrate 1 in this proposed design. Both of the designs in this work and in Ref. [18] take the lightweight merit of metallic cylinders, rather than introducing heavy dense dielectric substrates. Although the profile height of the proposed antenna array is a little bit larger than that in Refs. [2,3,18], the proposed array is suitable to be flush-mounted within a cavity without any radiation performance degradation. Moreover, this feature also avoids any protrusion out of the original airframe of an airborne platform. Finally, the unique narrow width characteristic makes the proposed monopole antenna possible for further phased antenna array development for beam scanning purposes.
5 Conclusions
In this paper, a wideband, end-fire array antenna embedded in a cavity is proposed. The antenna element and antenna array are fabricated and the performance is experimentally validated. It is the first UHF log-periodic end-fire antenna array with vertically polarized radiation that reported so far. The simulation indicates that the antenna array possesses a wide scanning range of ±45° in the horizontal plane over an operating UHF band of 300 MHz−900 MHz (100% bandwidth) for active VSWR < 2.0. A satisfactory agreement is obtained between simulated and measured radiation patterns in the fabricated 1×2 linear array. Together with its cavity embedded feature, the radiation performance demonstrates that this design is attractive for airborne platform applications that are used to search, discover, identify, and scout the aerial target, which is in the same horizontal plane with vertical or linear polarization. Moreover, a design concept is presented that potential applications or industries could benefit from this advancement.
Disclosures
The authors declare no conflicts of interest.