I. INTRODUCTION
Since the discovery of the positron,1 much attention has been devoted to the study of positron sources and their applications in various areas,2,3 including fundamental physics, medicine, and industry. Compared with conventional positron sources, laser-driven sources have many potential advantages, such as the ability to produce ultrashort bunches, at high energy, high density, and high yield. At present, by using high-power intense lasers, multi-MeV positrons can easily be produced in the laboratory.4–7 However, more energetic (i.e., in the GeV and TeV ranges) positron jets with extremely high density are still out of experimental reach and occur only in energetic astrophysical environments,2,8,9 such as γ-ray bursts, pulsars, and black holes. It would be very difficult to achieve such positron sources on earth with current laser technologies or traditional methods.
On the other hand, several laser facilities that are currently under development10–13 will deliver laser pulses with ultrahigh intensity of order 1023–1024 W/cm2 and power in the range 10–200 PW. This should open up a new realm of possibilities for light–matter interactions in the radiation and quantum-dominated regimes.14–17 For these proposed schemes, it has been shown that when the laser intensity is above 1023 W/cm2, there will be significant production of dense high-energy positron sources via the multiphoton Breit–Wheeler (BW) process18 from various media, such as plasmas19–25 and relativistic electron beams.26–28 However, the dense positron jets and bright γ-ray flashes that have been produced so far often exhibit large divergences.
In this paper, we present a practical approach to generate collimated GeV positron beams and bright γ-ray flashes at an achievable laser intensity of ∼1022 W/cm2 by using a plasma channel. Compared with the case of a uniform plasma slab, there is a significant improvement in collimation. It is also shown that the positron beams and γ-ray flashes all have durations of a few hundred attoseconds. Such atto-beams of relativistic particles and X/γ rays are of interest in diverse scientific and technological applications.29–32
II. NUMERICAL SIMULATION RESULTS
Figure 1(a) shows a sketch of our scenario. Two 10 PW laser pulses are incident on a near-critical-density (NCD) plasma channel from two sides and propagate along the x direction [see Fig. 1(b)], where the channel density profile is radially symmetric. During the laser propagation, the pulse intensity is greatly enhanced in the plasma channel. Meanwhile, dense attosecond electrons are trapped by the intense pulse and accelerated to multi-GeV energies, so that energetic attosecond γ rays are efficiently emitted via nonlinear Compton scattering (NCS).32,33 The dense GeV atto-beams of electrons and γ rays then collide with the second probe pulse from the right side, and a multiphoton BW process is triggered, resulting in abundant dense GeV positrons with atto-scale beam duration. As a comparison, we also consider a plasma with a uniform density distribution, as shown in Fig. 1(c).
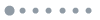
Figure 1.(a) Schematic of the generation of collimated GeV positron jets by the interaction of two 10 PW laser pulses with a plasma channel filled with NCD plasma. The drive pulse (laser #1) and probe pulse (laser #2) are incident from the left and right sides, respectively. Two cases are considered: (b) a plasma channel with a radially symmetric density profile and (c) a uniform plasma slab.
Three-dimensional (3D) particle-in-cell (PIC) simulations are performed using the code EPOCH,34 with both QED and collective plasma effects incorporated.35,36 In the simulations, two 10 PW-scale high-power linearly polarized Gaussian laser pulses (drive laser and probe laser) are incident with a time delay of from the left and right sides of the box, respectively. The temporal profiles of both laser pulses are trapezoidal with durations of () for the drive pulse and () for the probe pulse. The normalized amplitude of both lasers is , corresponding to an intensity of W/cm2, which is currently approachable in the laboratory,37 with a focus spot of . Here is the unit charge, is the electron mass, is the laser oscillation frequency, is the laser wavelength, and is the speed of light in vacuum. The NCD plasma has a transverse density profile in the plasma channel located between and , where is the critical density, , , and is the radial distance from the channel axis. For the case of a uniform plasma, the density is , to keep the total number of plasma electrons unchanged. The simulation box is , with a cell of and 16 macroparticles in each cell. To save computing resources, a moving window is employed at .
Figure 2(a) shows the energy spectra and the distributions of the electron energy density. It can be seen that electrons in the plasma channel can be accelerated to much higher energy than those in the uniform plasma. This can be attributed to the coupling effects of high-intensity laser interaction with NCD plasmas,38,39 where the plasma channel acts as a optical lens to enhance the intensity of a laser pulse significantly, as shown in Fig. 2(b). Since a large number of electrons are confined in the high-intensity region of the laser pulse, they consume the most laser energy by emitting high-energy γ rays during their rapid acceleration. It is interesting to note that the laser intensity is still enhanced fourfold within the plasma channel. The beam energy density of the accelerated electrons is as high as , with a high energy of 2.5 GeV and an ultrashort beam duration of several hundreds of attoseconds, which is more than eightfold higher than the threshold () for high-energy-density physics (HEDP).40 The interaction of such energetic electrons with the extremely intense laser fields results in , where is the critical parameter determining the importance of quantum-dominated radiation emission,24,36,41 as shown in Figs. 2(c) and 2(d). Here, is the electron Lorentz factor, is the electric field perpendicular to the electron velocity , and is the Schwinger field. During this process, the self-generated strong magnetic field plays an important role in enhancing . Finally, most of the drive laser pulse energy is absorbed by the plasma, and the electrons are accelerated to GeV energies, together with bright γ-ray emission. Such dense GeV atto-beams may open up new possibilities for a number of applications, providing ultrahigh-time-resolution studies with attosecond-scale resolution in various areas, such as high-energy physics, plasma physics, and astrophysics.
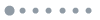
Figure 2.(a) Energy spectra of electrons at . (b) Transverse electric fields along the laser propagation axis. The insets in (a) and (b) show the distributions of the trapped electron energy density and laser transverse electric field, respectively, in the case of a plasma channel (top) and a uniform plasma slab (bottom). (c) and (d) show the distributions of the parameter along the x axis in the case of a plasma channel and a uniform plasma slab, respectively.
Since the critical parameter in the case of a plasma channel is much larger than in the case of a uniform plasma, GeV γ rays are efficiently emitted with a high photon energy density, as shown in Fig. 3(a). Here, the attosecond γ rays radiated have a smaller divergence angle at high photon energies than in the case of a uniform plasma slab, as can be seen in Figs. 3(b) and 3(c). With the plasma channel, the γ-ray beam has a total photon yield of at 25 MeV, a full width at half maximum (FWHM) cross section of , a FWHM divergence of , and a total pulse duration of ∼900 as at FWHM. The results indicate that GeV γ rays are obtained with a peak brightness of , which is several orders of magnitude higher than what is presently achievable in the laboratory42–45 and is also much brighter than the level obtained in other simulations.46–48 Meanwhile, the γ-ray beam is characterized by a desirable ultrashort duration of <370 as per pulse.
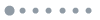
Figure 3.(a) Energy spectra of γ rays at . The insets show the energy density distribution of the γ-ray emission with a plasma channel (top) and a uniform plasma slab (bottom). (b) and (c) show the angular energy distributions of the emitted γ rays in the plasma channel and uniform plasma, respectively.
The greatly strengthened laser pulse accelerates the electrons to multi-GeV energies. During this process, extremely energetic γ-ray flashes are emitted via NCS in the NCD plasma. These accelerated electrons and emitted γ photons with GeV energies then collide with the probe laser pulse incident from the right side, triggering a multiphoton BW process. This can be described by the quantum parameter , where and are the energy and unit vector of the emitted photons.24,36,41 As a result, high-yield well-collimated GeV positron jets are effectively generated with an overdense density profile and attosecond-scale beam duration. Figure 4 shows the results of a 3D PIC simulation of positron generation by a plasma channel and by a plasma slab. It is clear that the jets can be significantly enhanced in the case of a plasma channel. The collimated GeV positron jets obtained have a high density of , with a small divergence angle of 14° and an ultrashort beam duration of 400 as at FWHM. The energy density of these positrons is about , which is -fold higher than the HEDP threshold. This offers an exciting new tool for positron-based research, potentially pushing such studies into the attosecond regime.
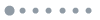
Figure 4.(a) Energy spectra of positrons from a plasma channel (red curve) and a uniform plasma slab (black curve), where the inset shows the positron angular divergence. (b) Evolution of total yield and energy of positrons with the interaction time, where the solid curves are for a plasma channel and the dashed curves are for a uniform plasma slab. (c) and (d) show the energy density distributions of positrons generated from a plasma channel and from a uniform plasma slab, respectively. The insets present the density profiles of positrons in the x–y cross section.
III. SCALING WITH LASER INTENSITY AND PLASMA CHANNEL LENGTH
The present simulations demonstrate a promising and efficient approach for generating well-collimated, energetic, dense positron jets with attosecond-scale beam duration from an NCD plasma channel, driven by 10 PW-scale intense lasers. To further explore the parametric effects and robustness of this scheme, a series of 3D PIC simulations are carried out employing different NCD plasma channels and laser intensities. First, the effect of laser intensity on the jet generation is investigated, with all other parameters being kept the same as before, except for the laser amplitude and the corresponding plasma density . Figure 5(a) shows the simulation results. It can be seen that as the laser intensity increases, the efficiency of laser production of positrons is enhanced significantly. This is due to the fact that with increasing laser intensity, it becomes easier to trigger the multiphoton BW process. Note that the critical quantum parameter . With forthcoming multi-PW laser facilities, our scheme has the potential for highly efficient generation of dense GeV positron jets and bright γ-ray flashes with the desirable attosecond-scale beam property.
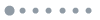
Figure 5.Yield , energy conversion efficiency , and cutoff energy of positrons as functions of (a) laser amplitude and (b) plasma channel length .
Figure 5(b) shows simulation results for plasma channel lengths ranging from to . It can be seen that a longer channel length improves the production of positrons. Both the maximum energy and the yield of positrons increase with , as does the efficiency of energy conversion from the laser pulse to the positrons. This is attributed to the accelerated electrons, which can reach a higher energy with a longer acceleration distance and efficiently radiate extremely energetic γ rays in NCD plasmas.38,39 However, further increases in channel length are not always desirable. For example, the generation of positrons saturates for , because the drive laser pulse is rapidly depleted in such a long plasma channel, so that both electron acceleration and γ-ray emission become limited and electron–positron pair production ceases to be enhanced. This effect could be used to tune and enhance positron jet generation in future experiments. It has been shown that the use of plasma and magnet devices49,50 may allow transport and focusing of relativistic positron atto-beams, although the underlying physics needs further study.
IV. SUMMARY
We have investigated the generation of collimated GeV attosecond positron beams from the interaction of 10 PW lasers with an NCD plasma at currently achievable laser intensities of . We have shown that high-yield, well-collimated, dense GeV attosecond positron beams are efficiently produced using a plasma channel. Compared with the uniform plasma case, the positron yield is greatly enhanced owing to strong focusing and subsequent intensity enhancement of the incident laser pulse in the plasma channel. The yield, energy conversion efficiency, and cutoff energy of the positrons obtained increase with increasing incident laser intensity, and further enhancement can be achieved by using a longer plasma channel. With the upcoming next-generation laser facilities (e.g., ELI,10 XCELS,11 Apollon,12 and SULF13), such collimated dense GeV positron jets and bright γ-ray flashes, both with desirable attosecond duration, may open up new avenues for ultrafast applications.