1. INTRODUCTION
Ultrafast fiber lasers (UFLs), which deliver pulses with extremely short durations (e.g., on the order of femtoseconds or picoseconds), have been proved as the most powerful tool for various and crucial applications such as strong-field physics, nonlinear optics, precision metrology, and ultrafine material processing. One distinguishing property of rare-earth-doped fibers is the large gain bandwidth (i.e., tens of nanometers), making possible the generation of ultrafast mode-locked laser pulses (). In order to achieve the mode-locking response, cavity longitudinal modes must be forced to lock together by either active electro-optic modulators or passive saturable absorbers (SAs). Comparatively, a passively mode-locked fiber laser using a real SA has the significant advantages of self-starting operation, low cost, high stability, and being maintenance-free.
Currently, the most prevalent absorber technology is the molecular beam epitaxy (MBE)-grown semiconductor SA mirror (SESAM) [1], which is widely applied in semiconductor lasers, UFLs, and solid-state lasers. However, SESAM has its own limitations, including long recovery time (), narrowband operation (), sophisticated fabrication, and a low damage threshold. Therefore, the pursuit of an ideal SA, the key module of a passively mode-locked fiber laser, has long been the goal of scientific researchers.
Two-dimensional (2D) materials, also denoted as atomic layered materials, define a new material morphology where single or few layers of atoms gather together in one direction, while in two other directions, they keep uniform and crystal-like expansions. With the reduction in physical dimension, 2D materials bring totally different energy band structures when compared to their bulk states, and possess unique optical and electronic characteristics [2–5] that have been employed in applications of microelectronics devices [6], biomedicine [7], energy [8], and chemistry [9]. As summarized in this review, one of the most exciting things is that they could be used as passive SAs, the mode-lockers for UFLs. The origin of saturable absorption in 2D materials is similar to SESAM, in that the absorption of injected light can be saturated under strong excitation due to the depletion of final states (i.e., Pauli blocking).
Sign up for Photonics Research TOC Get the latest issue of Advanced Photonics delivered right to you!Sign up now
Ignited by Bao et al. [10] and Sun et al. [11,12], who reported the initial UFLs mode-locked by graphene in 2009, a rapid exploration of the 2D material family as efficient fiber SAs has occurred. The most widely investigated 2D materials in this field are graphene [13,14], topological insulators (TIs) [15,16], transition metal dichalcogenides (TMDs) [17,18], and black phosphorus (BP) [19,20]. In addition, some newly emerging 2D materials continue to join the 2D family, like MXenes [21,22], bismuthene [23], and antimonene [24], all reported recently. The profusion of 2D materials together with diverse fiber integration methods brings quite a great flexibility in fabricating fiber SAs with unique and controllable parameters, allowing for broad bandwidth, ultrafast recovery, high damage threshold, low saturable influence, etc. In combination with rich rare-earth-doped fiber lasers in different wavelength regions, the development of UFLs mode-locked by 2D nanomaterials has been greatly facilitated over the last decade [25–29].
In this paper, we cover the state of art of UFLs mode-locked by 2D materials. In Section 2, a brief introduction to dominant 2D materials is made, including their atomic structures, energy band structures, linear and nonlinear absorptions, carrier lifetimes, etc. Related experimental approaches for fabricating these 2D materials and fiber SAs are also mentioned. In Section 3, the historical first demonstrations of UFLs mode-locked by 2D materials are presented. In Section 4, some important and interesting progress in recent years is summarized, mainly focusing on pulse width, repetition rate, and stability characteristics. In Section 5, new insights and current challenges regarding 2D materials are discussed.
2. 2D MATERIAL FAMILY FOR UFLs
In the fields of ultrafast photonics, 2D materials are characterized by their broadband saturable absorptions [30–32], ultrafast recovery [33–35], large nonlinear refractive indices [36,37], and potential as outstanding mode-lockers for UFLs. What follows is a brief overview of 2D materials’ atomic structures, bandgap structures, and recovery times. Table 1 shows a brief comparison of the 2D material family. Later in this section, corresponding material preparation and fiber integration techniques are also introduced.
A. 2D Materials
1. Graphene
Graphene, viewed as the pioneer of 2D materials, is a single layer of carbon atoms arranged in a 2D honeycomb lattice [14], facilitating great potential in the application of UFLs. Benefiting from its gapless Dirac cone [13], monolayer graphene is calculated to possess about 2.3% absorption of incident visible to infrared (IR) light. Graphene is special in that it enjoys ultrashort recovery time (), low saturable absorption ( [38]), great relative modulation depth ( per layer [10]), and wavelength-independent operation (ranging from the visible to the terahertz), allowing it to operate efficiently for the generation of broadband ultrafast laser pulses.
2. TIs
TIs define a new kind of material with nontrivial symmetry-protected topological order that behaves as insulators in their interior but whose surfaces contain gapless conducting states [15,16,50]. A small indirect bulk bandgap of 0.2–0.3 eV gives TIs a strong graphene-like broadband nonlinear response from the visible to the mid-IR. The lifetime of their phonon-induced carriers is short of several picoseconds, also making them useful for ultrafast light modulators. Three kinds of TIs, namely, , , and , are the most widely used TI SAs.
3. TMDs
TMDs are a class of more than 40 different semiconductors that share a formula of , where stands for a transition metal (e.g., Mo, W, Ti, Nb) and stands for a chalcogen (e.g., S, Se, or Te) [51,52]. In a TMD monolayer, the single transition metal layer is sandwiched between the two chalcogen layers, showing graphene-like layered structure. As for different monolayer TMDs, they have energy bandgaps varying from 1 to 2.5 eV. Surprisingly, the subbandgaps created by the edge states of TMDs allow efficient absorptions of light with photon energy much lower than their normal bandgaps [53,54]. Meanwhile, the short recovery times of TMDs are several picoseconds, which are also fast enough for ultrafast light modulation. For example, , , , and have been widely used for UFLs in 1.5 and 2 μm wavelength regions.
4. BP
BP is a thermodynamically stable allotrope of phosphorus at room temperature [19,55]. Like graphene, each phosphorus atom in BP is connected to three adjacent phosphorus atoms, forming a stable six-atom linked ring structure. The difference is that the BP structure is puckered, which reduces its symmetry and brings an angle-dependent nonlinearity [56]. It has tunable direct bandgaps varying from 0.35 eV (bulk) to 2 eV (monolayer), indicating its broadband nonlinear response deep into the mid-IR [57,45], which has been widely used for UFLs as well [58,59]. Research shows that nanosheet BP has a wavelength-dependent recovery time of 0.36 to 1.36 ps excited with photon energies from 1.55 to 0.61 eV [60]. It should be noted that BP would be oxidized when exposed in ambient conditions and needs encapsulation for scaling its long-term stability [61].
5. MXenes
MXenes represent a new class of 2D transition metal carbides, carbonitrides, or nitrides whose chemical formula is . Here, stands for transition metals (Sc, Ti, Zr, Hf, V, Nb, Ta, Cr, Mo, etc.), is carbide and/or nitride, and represents surface terminations (F, O, OH, etc.) [22,62]. Few-layer has an indirect energy bandgap of and a low absorption of . Stacking of 2D MXene materials generally occurs through van der Waals interaction without internal surface termination, as in the cases of graphene, phosphorene, and TMDs. A recent study showed that the main features observed in MXene monolayers were well conserved in stacked ones [47], indicating that well-functioning fiber SAs could be made using MXenes, thus avoiding the troublesome processes of monolayer dispersion.
6. Bismuthene
Due to its distinct electronic and mechanical properties, as well as its strong stability, bismuthene has attracted tremendous research interest [23,48]. Recent research shows that the layer-dependent optical bandgap of beta-bismuthene ranges from almost 0 to 0.55 eV, suggesting that it is a promising broadband optical material from the near-IR to the terahertz regions [24]. The short recovery time of bismuthene is 2.8 ps, which also indicates it is a potential ultrafast SA.
7. Other Materials
The aforementioned 2D materials offer distinct, yet complementary properties and hence, new opportunities for optical applications in UFLs [63–65]. But these are not enough, since better SAs with enhanced optical properties, such as much shorter carrier lifetimes, higher damage thresholds, and larger modulation depths are always desired. On the one hand, explorations for new 2D materials will never stop. On the other hand, modification of existing materials also provides an opportunity. The possibility of combining different 2D materials to form van der Waals heterostructures offers an exciting prospect for a wide range of new engineerable photonic devices [66,67]. This overcomes the intrinsic drawbacks of single materials, while enhancing performance greatly [68,69]. Recently, several UFLs mode-locked by heterostructure SAs have been reported [70,71].
B. Preparation and Characterization of 2D Materials
To summarize, there are many physical or chemical techniques used to obtain 2D materials, which can be classified into two categories, namely, top-down exfoliation and bottom-up growth [72]. Typical approaches regarding exfoliation are mechanical exfoliation (ME) [73], liquid-phase exfoliation (LPE) [74], and ion-intercalation exfoliation [75]. Meanwhile, chemical vapor deposition (CVD) [76], pulsed laser deposition (PLD) [77], pulsed magnetron sputtering (PMS) [78], and MBE [28] are representative growth techniques.
At the same time, plenty of measurement techniques have been introduced to characterize 2D materials. To analyze the atomic composition and structural characteristics, the X-ray diffractometer, Raman scattering spectroscopy, and photoluminescence measurements are often used. Scanning electron microscopy (SEM), atomic force microscopy (AFM), and atomic resolution scanning transmission electron microscopy (STEM) are used to characterize the morphology. With Z-scan or P-scan measurements, it is possible to measure the third-order nonlinear coefficients and saturable absorptions directly. Pump–probe spectroscopy is used to analyze the carrier lifetime. Recently, both Z-scan [36] and pump–probe spectroscopy [79] have been shifted to mid-IR regions. Micro pump–probe spectroscopy has also been promoted as an efficient tool to investigate 2D materials’ carrier transportation on the scale of micrometers [80,81].
C. Fiber Integration with 2D Materials
To fabricate SAs for all-fiber mode-locked UFLs, 2D materials must be transferred [82] or deposited [83] onto optical fibers, achieving sufficient interaction with intracavity laser light. Meanwhile, 2D materials could be mounted onto a transparent plate or a high-reflectivity mirror. In these cases, UFLs with non-all-fiber formats could also be established with free-space couplings. Both polarization-maintaining (PM) and non-PM fibers could be used for building these UFLs with 2D materials. Figure 1 shows some popular fiber coupling schemes. Briefly, these couplings could be summarized into two kinds of schemes: transmission coupling [Figs. 1(a)–1(c)] and evanescent-wave coupling [Figs. 1(d)–1(g)].
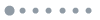
Figure 1.Fiber integration with 2D materials. (a)–(c) Transmission coupling; (d)–(g) evanescent-wave coupling; 2D materials are deposited or transferred on (a) fiber ends, (b) transparent plate, (c) reflection mirror, (d) tapered fiber, (e) side-polished fiber, (f) photonic crystal fiber, and (g) cladding-etched fiber.
1. Transmission Coupling
As shown in Fig. 1(a), the most common transmission coupling sandwiches SA materials between two fiber ends directly. Large area uniform 2D materials like ME-prepared graphene, CVD-grown graphene or TMDs, and MBE-grown TIs could be easily integrated with transferring methods [82]. Monolayer or few-layer nanosheet materials embedded in thin organic polyvinyl alcohol (PVA)/polymethyl methacrylate (PMMA)/polydimethylsiloxane (PDMS) films could be sandwiched too. For nanosheets in solutions, direct optical heat deposition to a fiber end is often used. In general, these methods are simple, and commercial fiber connector/physical contact (FC/PC) or fiber connector/angled physical contact (FC/APC) could be used directly. Figures 1(b) and 1(c) depict the cases when 2D materials are transferred or deposited onto a transparent plate or a high-reflectivity mirror, respectively. Besides, discrete optical components such as lenses must be adopted, resulting in free-space coupling with fibers. It should be noted that transmission coupling does have its disadvantages, such as a low damage threshold.
2. Evanescent-Wave Coupling
To scale the damage threshold of fiber SAs, evanescent-wave coupling usually provides a better choice. In this condition, the fiber-guided laser light in the core originally would leak out and interact with 2D materials on the side. The main four evanescent-wave couplings are sketched in Fig. 1, including tapered fiber [84], side-polished fiber [85], photonic crystal fiber [86], and cladding-etched fiber [87]. Both side-polished fiber [88] and photonic crystal fiber [89] have already been demonstrated to support high-power mode-locking operations.
After integration, a fiber SA component should be characterized further with methods including linear transmission spectroscopy and fiber-balanced twin-detector measurement [90], reflecting the fiber SAs’ modulation depths and nonsaturable loss. These tests are always important and indispensable because they reflect whether the integration process has been successful or not.
3. FIRST DEMONSTRATIONS OF UFLs MODE-LOCKED BY 2D MATERIALS
As discussed in Section 2, 2D materials are innate broadband SAs, enabling the realization of mode-locked UFLs at luxuriant wavelengths. The numerous laser transitions available from trivalent rare-earth ions like , , , and lend them the ability to generate light over a wide selection of wavelengths [91–94]. Despite different kinds of fibers, fibers made of silica and fluoride (, ZBLAN) glasses are the most widely used. Figure 2 sketches the very initial demonstrations of 2D materials-based UFLs at 1.0, 1.5, and 2.0 μm (silica), and 3.0 μm (ZBLAN) over the last decade.
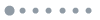
Figure 2.First demonstrations of UFLs mode-locked by 2D materials at different wavelengths.
Bao et al. reported the first graphene -doped fiber laser (EDFL) at 1550 nm [10] in 2009. From Fig. 3(a), one can see that a ring-cavity EDFL was mode-locked by a CVD-grown graphene film sandwiched between two fiber ends. In this case, a stable and regular soliton pulse train was generated, along with a repetition rate of 1.79 MHz and output power of 2 mW. The output pulses had a temporal width of 756 fs and a spectral bandwidth of 5 nm, indicating the ultrafast characteristics. Soon after that, mode-locked pulses at 1576.3 nm with a pulse width of 415 fs and a repetition rate of 6.84 MHz were obtained by Zhang et al. from a dispersion-managed cavity fiber laser [95]. At the end of 2009, Sun et al. also demonstrated their first work on an ultrafast EDFL mode-locked by monolayer and few-layer graphene flakes [12]. They proposed that high-performance ultrafast pulses could also be obtained with a graphene–PVA composite fabricated by using wet-chemistry techniques. These works in 2009 were pioneering, opening the door for UFLs mode-locked by 2D materials.
![CVD-grown graphene mode-locked EDFL [10]. (a) Laser configuration; (b) output pulse train; (c) output laser spectrum; (d) autocorrelation trace. Reproduced with permission, Copyright 2009, Wiley-VCH.](/Images/icon/loading.gif)
Figure 3.CVD-grown graphene mode-locked EDFL [10]. (a) Laser configuration; (b) output pulse train; (c) output laser spectrum; (d) autocorrelation trace. Reproduced with permission, Copyright 2009, Wiley-VCH.
As aforementioned, it is feasible to transplant mode-locking operation to other wavelengths, since gapless graphene makes it a natural broadband SA. Just one year later, Zhao et al. reported an ultrafast -doped fiber laser (YDFL) mode-locked by a few-layer CVD-grown graphene film. They obtained dissipative soliton pulses at 1069.8 nm with a spectral bandwidth of 1.29 nm and a pulse width of 580 ps [96]. In 2012, Zhang et al. reported the first graphene–PVA composite mode-locked -doped fiber laser (TDFL) at 1940 nm [97]. The laser output pulses had temporal widths of 3.6 ps and a pulse energy of at a repetition rate of 6.46 MHz. UFLs in this wavelength region are important because of eye-safe operation and their potential as laser scalpels. In 2015, a graphene mode-locked -doped fiber laser (HDFL) at 2107 nm with a pulse width of 1.8 ps was further demonstrated [98].
In the meantime, researchers also paid much attention to other kinds of 2D materials [3,72]. TIs, TMDs, BP, MXenes, and bismuthene were successively fabricated as fiber SAs and applied into mode-locked UFLs. To the best of our knowledge, the first demonstration of UFLs mode-locked by was in 2012 [99], in 2014 [100], BP in 2015 [101], MXene [47] and bismuthene [102] in 2017. Interestingly, almost all these initial demonstrations were at 1.5 μm except at 1 μm [100], which might benefit from the telecommunications boom. Meanwhile, these 2D materials were also investigated to explore the broadband lasing wavelengths like graphene [103–108]. In summary, not only graphene, but also TIs, TMDs, and BP have been used for the generation of 1, 1.5, and 2.0 μm ultrafast pulses in these corresponding silica fiber lasers. Note that these demonstrations of broadband SA characteristics were indirect because 2D materials were prepared by different groups, even with different methods. In 2014, Fu et al. put forward a direct demonstration of graphene’s broadband saturable absorption by inserting a single-fiber SA into three codoped fiber lasers: YDFL, EDFL, and (THDFL), respectively [30]. Thus,mode-locked pulses with center wavelengths of 1035, 1564, and 1908 nm were achieved.
Since graphene, Tis, and BP are gapless or have small energy bandgaps, as introduced in Section 2, they could also be used for mid-IR mode-locking operations. However, silica fiber does not work in the mid-IR due to its huge absorption. The adoption of fluoride ZBLAN fibers for generating ultrafast pulses opens up another playground in the mid-IR for the nonlinear studies of 2D materials. But due to the lack of mid-IR fiber components, current mid-IR UFLs had to adopt non-all-fiber formats limited by a free-space coupling scheme.
In 2015, Yin et al. designed the first mid-IR UFL mode-locked by 2D materials [109]. The linear-cavity UFL adopted a piece of -doped ZBLAN fiber as the gain fiber and a high-reflection gold mirror covered with nanosheets as the SA mirror. The output pulses had a repetition rate of 10.4 MHz, a pulse width of , and a center wavelength of 2830 nm. Soon after that in 2015, Zhu et al. also reported the first mid-IR graphene mode-locked -doped ZBLAN fiber laser at 2.78 μm [110]. A CVD-grown four-to-six layer graphene was transferred onto a gold mirror as the SA. The output had a pulse width of 42 ps and a repetition rate of 25.4 MHz. Few-layer BP SA mirrors have also been built for mid-IR mode-locked UFLs since 2016 [111,112]. In 2016, Qin et al. transferred mechanically exfoliated BP flakes onto a gold mirror as a BP SA mirror [111]. The excellent performance of the BP SA mirror in the mid-IR brought stable mode-locked pulses at 2783 nm with a maximum output power of 613 mW, a repetition rate of 24 MHz, and a pulse width of 42 ps. A recent work showed that BP could even be used to modulate lasing at 3489 nm in an -doped ZBLAN fiber laser [112], resulting in a stable mode-locked pulse train with a repetition rate of 28.91 MHz.
4. SIGNIFICANT PROGRESS OF UFLs MODE-LOCKED BY 2D MATERIALS
Most of these aforementioned initial UFLs mode-locked by 2D materials are proof-of-concept demonstrations. In fact, their lasing performances are hard to satisfy diverse applications. Therefore, more crucial parameters like SA modifications, cavity dispersion and nonlinearity management, coupling ratio, and gain adjustments are often adopted to improve laser performance. In the following, representative high-performance UFLs mode-locked by 2D materials are reviewed from three aspects: toward shorter pulse widths, higher repetition rates, and better stabilities. Due to the immature ZBLAN fiber components [113], high-performance mode-locked mid-IR fiber lasers are rare. Therefore, we focus on silica fiber-based UFLs in this section.
A. Short Pulse Width
The output pulse width of a mode-locked fiber laser depends mainly on its spectral bandwidth and chirp [114]. In fact, the broadband saturable absorption feature of 2D materials could modulate all longitude modes within the whole gain bandwidth of rare-earth ions, leading to desirable ultrafast pulses. When the laser cavity operates in the anomalous dispersion regime, mode-locked pulses can easily evolve into femtosecond solitons, considering the dispersion is comparable to nonlinearity [115].
Based on numerical simulations, Jeon et al. found that in a mode-locked UFL, the adoption of SAs with larger modulation depth could induce the temporal shortening of output pulses [116]. Considering that monolayer 2D materials’ absorption is low, the problem is how to improve the modulation depth of a 2D material SA. In 2015, Sobon et al. put forward a stacking method for monolayer graphene to achieve the desired number of layers [117]. They demonstrated that by increasing the layer numbers, the graphene SA’s modulation depth increased, and the output pulse width decreased. This idea of engineering modulation depth by modifying layer numbers was soon adopted in other kinds of 2D materials like [118] and [119].
In order to shorten the mode-locking pulse width, dispersion management is also indispensable. By adopting gain and passive fibers with opposite dispersions [120] or inserting a dispersion compensation grating [121], the net cavity dispersion could be minimized. Indubitably, external pulse compression is a promising alternative. A fiber chirp pulse amplification (CPA) chain can not only scale up pulse energy, but also induce broadening of the amplified spectrum, leading to a much shorter pulse width after compression. Representative results of UFLs mode-locked by 2D materials at short pulse widths are presented in Table 2.

Table 2. UFLs Mode-Locked by 2D Materials with Short Pulse Widths
Table 2. UFLs Mode-Locked by 2D Materials with Short Pulse Widths
Laser Configuration | SA | Pulse Width | Wavelength | Spectral Bandwidth | Ref. | Dispersion compensated ring-cavity EDFL | 60-layer CVD-grown graphene | 88 fs | 1545 nm | 48 nm | [122] | Ring-cavity TDFL | 24-layer CVD-grown graphene | 603 fs | 1940 nm | 6.6 nm | [123] | Dispersion compensated ring-cavity HDFL | Few-layers graphene | 190 fs | 2059 nm | 53.6 nm | [124] | Linear-cavity Er3+-doped ZBLAN fiber laser | 4–6 layer CVD-grown graphene | ∼42 ps | 2784.5 nm | 0.21 nm | [110] | Ring-cavity EDFL + EDFA | 30-layer CVD-grown graphene | 224 fs/24 fsa | 1560 nm | 11.5 nm/136 nmb | [125] | Ring-cavity TDFL + TDFA | 12-layer CVD-grown graphene | 656 fs/260 fsa | 1968 nm | 9.4 nm/15 nmb | [126] | Ring-cavity YDFL | 250 nm PMS-deposited TI−Bi2Te3 | 5.3 ps | 1036.7 nm | 8.28 nm | [78] | Ring-cavity EDFL | PLD-prepared TI−Sb2Te3 | 70 fs | 1542 nm | 63 nm | [127] | Ring-cavity EDFL | Bulk-structured TI−Bi2Te3 | 795 fs | 1935 nm | 5.64 nm | [104] | Linear-cavity Ho3+-doped ZBLAN fiber laser | LPE-prepared TI−Bi2Te3 | 6 ps | 2830 nm | 10 nm | [109] | Ring-cavity EDFL | CVD-grown TMD−WSe2 | 163.5 fs | 1557.4 nm | 25.8 nm | [128] | Ring-cavity EDFL | PLD-prepared TMD−WS2 | 67 fs | 1540 nm | 114 nm | [129] | Ring-cavity EDFL | LPE-prepared BP | 102 fs | 1555 nm | 40 nm | [130] | Ring-cavity TDFL | ME-prepared BP | 739 fs | 1910 nm | 5.8 nm | [108] | Linear-cavity Ho3+-doped ZBLAN fiber laser | LPE-prepared BP | 8.6 ps | 2866.7 nm | 4.35 nm | [131] | Ring-cavity EDFL | LPE-prepared bismuthene | 193 fs | 1561 nm | 14.4 nm | [132] | Ring-cavity EDFL | MXene-Ti3C2Tx | 159 fs | 1555 nm | 22.2 nm | [133] |
|
The shortest pulse width (88 fs) output directly from a graphene mode-locked EDFL was reported by Sotor et al. in 2015 [122]. A 60-layer CVD-grown graphene/PMMA composite was sandwiched between two fiber ends as an SA. With dispersion compensation, the ring-cavity EDFL had a net dispersion of , resulting in a stretched mode-locking operation at 1545 nm with a spectral bandwidth of 48 nm. Also utilized with CVD-grown graphene films, shortest pulse widths of 603 fs at 1940 nm [123] and 190 fs at 2059 nm [124] were reported. However, due to the lack of dispersion engineering, the graphene mode-locked ZBLAN fiber laser at 2784.5 nm had a long pulse width of 42 ps [110].
With all-fiber CPA systems, ultrafast pulses shorter than 24 and 260 fs at 1560 and 1968 nm were obtained [125,126], respectively. Figure 4 shows the corresponding result when the shortest pulse width of 24 fs was realized [125]. As presented in Fig. 4(a), the laser system consisted of only two types of PM fibers, ensuring simplicity and stability. A 30-layer graphene/polymer composite as described in detail in Ref. [117], was used as the fiber SA in the mode-locked EDFL. The output pulse from the oscillator had the shortest temporal width of 224 fs, associated with a lasing wavelength of 1560 nm and a spectral bandwidth of 11.5 nm. After amplification with an -doped fiber amplifier (EDFA) and compression in the gain fiber, the fiber laser system delivered few-cycle optical pulses with a pulse width short of 24 fs and a spectral bandwidth of 136 nm.
![Graphene mode-locked EDFL that delivers 24 fs pulses [125]. (a) Laser configuration; (b) optical spectrum; (c) autocorrelation trace; (d) measured RF spectrum. Reproduced with permission. Copyright 2016, IOP Publishing.](/Images/icon/loading.gif)
Figure 4.Graphene mode-locked EDFL that delivers 24 fs pulses [125]. (a) Laser configuration; (b) optical spectrum; (c) autocorrelation trace; (d) measured RF spectrum. Reproduced with permission. Copyright 2016, IOP Publishing.
As illustrated in Table 2, one can acquire the shortest output pulse widths for the UFLs mode-locked by other kinds of 2D materials. These results are generated directly from mode-locked laser oscillators without amplification and compression. In the 1.5 μm wavelength region, the shortest output pulses mode-locked by TIs, TMDs, BP, bismuthene, and MXenes were 70 fs [127], 67 fs [129], 102 fs [130], 193 fs [132], and 159 fs [133], respectively. These works demonstrated that other 2D materials also had the ability to generate ultrafast laser pulses. Comparatively, the output pulses at other wavelengths like 1 and 2 μm are still much longer than pulses at 1.5 μm, indicating much more work is required to shorten pulses in the future. Meanwhile, robust fiber amplifiers operating in these wavelength ranges are also required to scale up pulse energy and peak power, and further shorten the pulse width.
B. High Repetition Rate
The pursuit of higher repetition rates is another important aspect. In particular, lasers with repetition rates from several to hundreds of gigahertz are important for high-speed optical communication systems and microwave generation. The short recovery times of 2D materials make them good candidates for gigahertz pulse generation.
Table 3 shows the representative results of high repetition rate UFLs mode-locked by 2D materials. For fundamental mode-locking operation, the repetition rate of the pulse train is the same as the longitudinal mode spacing, whose increment can only be achieved by reducing cavity length. In 2012, Martinez et al. reported a graphene mode-locked EDFL with a linear cavity, whose pulse repetition rate was 9.67 GHz [134]. In 2015, Wu et al. also demonstrated a -based short-cavity EDFL at a fundamental repetition rate of 463 MHz [139]. However, due to the fiber gain limitation, short-cavity configuration is difficult to improve the repetition rate further (e.g., ).

Table 3. UFLs Mode-Locked by 2D Materials with High Repetition Rates
Table 3. UFLs Mode-Locked by 2D Materials with High Repetition Rates
Laser Configuration | SA | Repetition Rate | Ref. | ∼1 cm short linear-cavity EDFL | LPE-prepared graphene | 9.67 GHz | [134] | 21st-harmonic mode-locked ring-cavity EDFL | ME-prepared multilayer graphene | 2.22 GHz | [135] | Ring-cavity EDFL with FP filter | LPE-prepared graphene | 100 GHz | [136] | Ring-cavity EDFL with microfiber knot filter | LPE-prepared graphene | 106.7 GHz | [137] | Ring-cavity YDFL with microfiber knot filter | LPE-prepared graphene | 162 GHz | [137] | 418th-harmonic mode-locked ring-cavity EDFL | LPE-prepared TI−Bi2Te3 | 2.04 GHz | [138] | 170th-harmonic mode-locked ring-cavity EDFL | PLD-prepared TI−Bi2Te3 | 2.95 GHz | [77] | ∼22 cm short linear-cavity EDFL | LPE-prepared TMD−MoS2 | 463 MHz | [139] | 212th-harmonic mode-locked ring-cavity EDFL | LPE-prepared TMD−MoSe2 | 3.27 GHz | [140] | 10th-harmonic mode-locked ring-cavity HDFL | LPE-prepared BP | 290 MHz | [141] |
|
By inserting a comb filter like Fabry–Perot (FP) filter or microknot filter into a fiber laser cavity to form a composite cavity, it is also possible to increase output repetition rate by filtering out some longitudinal modes [114]. In 2015, Qi et al. reported the generation of a 100 GHz pulse train in an EDFL by using a graphene tapered fiber SA and an FP filter [136]. Figure 5 shows a recent work by Liu et al. in which a graphene-deposited microfiber knot filter was used in ring-cavity fiber lasers, providing the spectral filtering and saturable absorption effect [137]. When the filter was implemented into a YDFL, a pulse train at 1 μm with a 162 GHz repetition rate was generated. When it was inserted into an EDFL, a pulse train in the 1.5 μm region with a 106.7 GHz repetition rate was addressed.
![Hundred gigahertz repetition rate graphene mode-locked UFLs [137]. (a) Laser configuration; (b) graphene microfiber knot filter; (c) laser spectrum at 1 μm; (d) laser spectrum at 1.5 μm; (e) autocorrelation trace at 1 μm; (f) autocorrelation trace at 1.5 μm. Reproduced with permission. Copyright 2018, OSA Publishing.](/Images/icon/loading.gif)
Figure 5.Hundred gigahertz repetition rate graphene mode-locked UFLs [137]. (a) Laser configuration; (b) graphene microfiber knot filter; (c) laser spectrum at 1 μm; (d) laser spectrum at 1.5 μm; (e) autocorrelation trace at 1 μm; (f) autocorrelation trace at 1.5 μm. Reproduced with permission. Copyright 2018, OSA Publishing.
The harmonic mode-locking technique is an alternative important approach to realize high repetition rate outputs. In 2012, a graphene-based 21st-harmonic mode-locked fiber laser was reported by Sobon et al., with a pulse repetition rate of 2.22 GHz [135]. Other kinds of 2D materials like TIs, TMDs, and BP are also efficient for achieving harmonic mode-locking operations. With a fiber SA implemented in a ring-cavity EDFL, Luo et al. raised the repetition rate to 2.04 GHz, corresponding to a high harmonic order of 418 [138]. Another work by Yan et al. also utilized a fiber SA in a ring-cavity EDFL, but with a higher fundamental repetition rate of 200 MHz. In this case, a harmonic mode-locking repetition rate of 2.95 GHz was obtained at a 170th-harmonic order [77]. To date, the highest repetition rate of a TMD mode-locked UFL was accomplished by Koo et al. in 2016 [140]. In their work, a composite depositing side-polished fiber was incorporated as an SA within a ring-cavity EDFL, where a repetition rate up to 3.27 GHz at a harmonic order of 212 was obtained. Considering various applications of high repetition rate pulses, we anticipate that high repetition rate UFLs mode-locked by 2D materials will continue to be a hot topic in the future.
C. High Stability
Many applications of UFLs, such as optical frequency combs [142,143] and pure microwave generation, are in great need of highly stable ultralow-noise laser pulses. Therefore, the stability of a UFL is always the first consideration. A simple measurement to reflect the stability is to check the RF spectrum of pulse trains. If a mode-locked fiber laser has a high stability with RF harmonics extending to several gigahertz, it is regarded as an ideal device for gigahertz signal generation. Some representative results of UFLs mode-locked by 2D materials are summarized in Table 4.

Table 4. Highly Stable UFLs Mode-Locked by 2D Materials
Table 4. Highly Stable UFLs Mode-Locked by 2D Materials
Laser Configuration | SA | SNR at frep | RF Resolution | RF Spanning | Ref. | Ring-cavity EDFL | LPE-prepared graphene | 87.4 dB | 10 Hz | – | [120] | Ring-cavity EDFL | CVD-grown graphene | 70 dB | – | 0–10 GHz | [144] | Ring-cavity TDFL | CVD-grown graphene | 75 dB | 33 Hz | 0–3.6 GHz | [126] | Ring-cavity EDFL | PMS-deposited TI−In2Se3 | 90 dB | 30 Hz | 0–1.5 GHz | [145] | Ring-cavity EDFL | PMS-deposited TMD−MoTe2 | 93 dB | 10 Hz | 0–1 GHz | [146] | Ring-cavity TDFL | PMS-deposited TI−Sb2Te3 | 84 dB | 10 Hz | 0–1 GHz | [147] | Ring-cavity TDFL | PMS-deposited TI−Wte2 | 95 dB | 10 Hz | 0–0.5 GHz | [148] | Ring-cavity EDFL | CVD-grown TMD−WSe2 | 96 dB | 20 Hz | 0–0.6 GHz | [128] |
|
In 2010, Popa et al. demonstrated a highly stable graphene mode-locked EDFL [120]. The ring-cavity laser had a net dispersion of . A large RF signal-to-noise ratio (SNR) of 87.4 dB was realized at the fundamental repetition rate of 27.4 MHz. In 2014, Sobon et al. reported a high-power fiber CPA laser system at 1560 nm [144]. A highly stable CVD-grown graphene mode-locked EDFL was selected to provide seed pulses. It had an RF SNR of 70 dB at the repetition rate of 50 MHz, whose RF spectrum exhibits a slight decay compared to the case of 0–10 GHz, implying its high stability. Other 2D materials like TIs and TMDs have also been used for generating low-noise mode-locked UFLs. Yan et al. used the PMS technique to deposit TI and TMD films onto fiber tapers for fabricating high-performance fiber SAs. By inserting these fiber SAs into ring-cavity EDFLs [145,146] and TDFLs [147], stable mode-locked pulse trains with ultrahigh SNRs on RF spectra were obtained. In 2018, Liu et al. prepared a CVD-grown TMD- film and transferred it onto a tapered fiber to fabricate a fiber SA. They demonstrated a highest SNR of 96 dB at a pulse repetition rate of 63.133 MHz with a ring-cavity EDFL [146].
Figure 6 depicts the measured optical spectra and RF spectra of these representative results. As can be seen from the optical spectra, these highly stable laser pulses were all typical soliton pulses mode-locked in anomalous regimes. To further characterize the stabilities of mode-locked UFLs, relative intensity noise and time jittering should be taken into account. In the future, we hope that more techniques and methods will be used to suppress lasing noise and enhance long-term stability.
![Highly stable UFLs mode-locked by 2D materials. (a)–(c) Laser spectra; (d)–(f) measured RF spectra. (a) and (d), Ref. [146]; reproduced with permission; copyright 2018, OSA Publishing. (b) and (e), Ref. [148]; reproduced with permission; copyright 2017, OSA Publishing. (c) and (f), Ref. [128]; reproduced with permission; copyright 2018, IOP Publishing.](/Images/icon/loading.gif)
Figure 6.Highly stable UFLs mode-locked by 2D materials. (a)–(c) Laser spectra; (d)–(f) measured RF spectra. (a) and (d), Ref. [146]; reproduced with permission; copyright 2018, OSA Publishing. (b) and (e), Ref. [148]; reproduced with permission; copyright 2017, OSA Publishing. (c) and (f), Ref. [128]; reproduced with permission; copyright 2018, IOP Publishing.
5. CHALLENGES AND FUTURE DIRECTIONS
According to previous discussions, there are indeed some aspects of 2D materials that are better than those of SESAMs, like excellent broadband saturable absorption and ultrashort recovery time. However, despite the current achievements, the lack of fine-controlled material fabrication still remains a hurdle to mass production of most of these 2D materials [133]. Maybe the industrialization of graphene is a great start. Besides, the long-term stability of these 2D materials should also be further exploited. Methods used for oxidation resistance [2], hydrolytic resistance [61], and suppression of photon-induced degradation should be fully considered as the next steps. We also think that the fabrication of a 2D material fiber SA with customized nonsaturable loss, modulation depth, and recovery time is of critical importance. It is likely that future work in this field will establish more systematic guidelines for SA design and optimization.
Obviously, there is abundant room for further progress in the pursuit of high-performance UFLs mode-locked by 2D materials. The broadband saturable absorption of 2D materials makes them good candidates for two-band mode-locking operations simultaneously. In 2014, Sotor et al. reported a passive synchronization of an EDFL and a TDFL enhanced by a common graphene SA [149]. It might help for few-cycle pulse generation with coherent combination or difference frequency generation in the mid-IR [150]. Meanwhile, the introduction of advanced optical techniques, such as time-lens [151], dispersive Fourier transform [152–154], and coherent sampling [155] measurements, to reveal the real-time pulse build-up dynamics of UFLs mode-locked by 2D materials is highly desirable. We believe that after getting insight into the buildup dynamics and making positive modifications to 2D materials-based SAs, many more high-performance UFLs will be put forward in the future. Although the main aim of this paper is to review the progress of UFLs mode-locked by 2D materials, we would like to point out the broadband 2D materials could also be exploited for novel photonic components, like photodetectors [156–160], sensors [161–164], active-optical modulators [165,166], and all-optical modulators [65,167–175]. Besides these, we also have confidence that more unprecedented ultralow-noise ultrafast mode-locked pulses or optical frequency combs will be realized with novel 2D materials-based SAs.
6. CONCLUSIONS
We have reviewed both initial and significant UFLs mode-locked by 2D materials in this paper. The intention of this review is to provide a brief survey of UFLs mode-locked by 2D materials in the past decade. Though it is not yet possible to be completely exhaustive, a number of important results have been covered, and future insights and challenges have been put forward.