1. INTRODUCTION
Since surface-enhanced Raman spectroscopy (SERS) was discovered in the 1970s [1], it has attracted a great deal of attention because it can perform non-destructive and high-sensitivity examination [2,3], and it can even provide the “fingerprint” information on a single-molecule level [4,5]. As an ultrasensitive and quantifiable analytical technique for examining unknown samples or distinguishing the target analytes from a mixture, SERS has been widely explored in fields of chemistry [6], biology [7], energy [8], materials [9], medicine [10], etc.
SERS mainly relies on the enhancement of the electric field near the metal nanostructures to increase Raman scattering intensity when the target analytes are attached to the nanostructures [11]. With the rapid development of nanofabrication technologies, such as E-beam/focused ion beam lithography [12,13], femtosecond processing [14], self-assembly [15–17], nanoimprinting [18], and chemical reactions [19], many kinds of metallic nanostructures, including nanorods [20], nanoholes [21], nanoflowers [22], nanostars [23], nanoisland films [24,25], etc., have been prepared for SERS applications. Evaluating the performance of a SERS substrate takes into consideration not only their sensitivity, uniformity, and reproducibility, but also the economics, convenience, and throughput of the preparation process [26]. Among them, the self-assembly method provides an alternative choice for preparing SERS substrates in an economical and convenient way [27] and for being of high SERS performance. So far, based on the improved self-assembly techniques, various metal nanostructures have been fabricated and used for SERS examination with high performance [28–32].
Sensitivity is a key criterion for evaluating the performance of SERS substrates, and it depends on the electric-field intensity of the “hot-spot” formatted near the nanostructures due to the localized surface plasmon resonance (LSPR) effect [33]. Since the morphology of the nanostructures plays an essential role in the LSPR effect and determines the electric-field intensity of the “hot-spot,” much effort has been devoted to preparing the metal nanostructures with special morphology for optimal electric-field enhancement. A gap mode constructed using two adjacent nanostructures with a nanogap can achieve stronger electric-field enhancement than the surface local mode near the apex of a nanostructure [34–38]. Nevertheless, the nanostructures with a gap mode are difficult to prepare with their characteristics of large scale and uniform and periodic arrangement. Instead, the fabrication of the nanostructures with surface local mode can meet these requirements with an economic and convenient method.
Sign up for Photonics Research TOC Get the latest issue of Advanced Photonics delivered right to you!Sign up now
Generally, the linear polarization beam (LPB) with Gaussian modal distribution has been widely used to excite the metal nanostructure to achieve SERS examination [39–41]. Due to the varied spatial symmetries of some metal nanostructures, the monotonic polarization distributions of LPB make it difficult to achieve optimal excitation of the surface plasmon modes. However, the azimuthal vector beam (AVB) used as the excitation source can obtain a better excitation effect than LPB excitation [42–46] because the azimuthal polarization distribution of the AVB has a richer polarization component perpendicular to the surface of the metal nanostructures. Therefore, it is possible that the AVB can be used to achieve better surface plasmon mode excitation than LPB, and thus it can obtain better SERS performance.
In this paper, a method for enhancing SERS performance of a silver triangular nanoprism array (ATNA) substrate vertically excited via a focused AVB is presented. The ATNA substrate is prepared based on the traditional self-assembled and modified film lift-off method. Based on the model established adopting the structural parameters of the ATNA substrates, theoretical calculation indicates that the AVB used as excitation source of ATNA substrates can achieve greater electric-field enhancement than LPB excitation. SERS sensitivity experimentally obtained via AVB excitation of an ATNA substrate is (1 M = 1 mol/L) using rhodamine 6G (R6G) as the target analyte, and it is 2 orders of magnitude lower than that of LPB excitation of . Furthermore, the uniformity and reproducibility of the ATNA substrates are also examined via Raman mapping and batch-to-batch measurement, respectively, and the Raman enhancement factor is estimated to be , revealing good SERS performance of the ATNA substrates.
2. METHODS
A. Experimental System of SERS Examination
In this experiment, the SERS performance of the ATNA substrate is examined by using a home-built Raman setup integrated with single point Raman spectrum measurement and scanning imaging via a Raman characteristic peak as shown in Fig. 1. A 632.8 nm laser with power of 23 mW is used as the excitation light. The Gaussian mode output from the single-mode fiber (SMF) is collimated as a parallel light via the micro-objective (). The power of the excitation light is adjusted via the attenuator (A), and the polarizer (P) is used to purify the LPB. The polarization direction of the LPB is rotated via the half-wave plate (HWP), and then it passes through the vortex plate (VP, WPV10L-633). When the polarization direction of the LPB is perpendicular to the fast axis of the VP, the LPB is converted to an AVB. The mode intensity distribution and polarization examination result of the AVB is shown in the inset in Fig. 1. The with a numerical aperture (NA) of 0.8 is used to focus the AVB on the surface of the ATNA substrate and collect the Raman spectrum. The Raman spectrum is coupled into the spectrometer via the lens () after filtering the Rayleigh scattering through the filter (F). A charge-coupled device (CCD) is used to observe the position of the focused spot on the surface of the ATNA substrate, under illumination of a white light source (WLS). A self-written LabVIEW program is used to simultaneously control the piezoelectric stage (PZS) and the spectrometer to enable point-by-point scanning imaging.
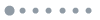
Figure 1.Sketch map of the experimental setup for SERS examination of the ATNA substrate. Inset is (a1) the mode intensity distribution and (a2)−(a4) the polarization examination results of the AVB.
B. Simulation Method
The wavelength of excitation light is set to . The numerical aperture (NA) of the micro-objective (MO) used in the SERS examination configuration is . The focused characteristics of LPB and AVB are accordingly calculated in the case of , based on the Richards–Wolf vector theory [47,48].
The 3D finite-difference time-domain software (FDTD, Lumerical) is adopted to calculate the electric-field intensity enhancement of the ATNA substrate excited via the focused LPB and AVB. The permittivity of Ag is taken from the experimental measurements of Johnson and Christy [49], and the perfectly matched layers (PMLs) are used as absorption boundaries to simulate the structures placed in an infinitely large free space. The focused LPB with calculated with FDTD software is used as the excitation source to calculate the electric-field enhancement characteristics of one nanoprism array unit. The MATLAB codes of the focused AVB with are written based on the Richards–Wolf theory, and then integrated into the FDTD software to calculate the electric-field enhancement.
3. RESULTS AND DISCUSSION
A. Fabrication and Characterization of the ATNA Substrates
The fabrication process of the ATNA substrate is shown schematically in Figs. 2(a)–2(c). A monolayer ordered hexagonally close-packed polystyrene (PS) nanosphere (PSN-00600, PSN-00400, PSN-00300, Tjdaekj, China) on the silicon wafer is first formed using the traditional Langmuir–Blodgett (LB) self-assembled method [50,51]. A 100 nm thick silver (99.99%, SANTE MATERIAL, China) film is deposited on the prepared close-packed PS nanospheres mask, as shown in Fig. 2(a), using the vacuum thermal evaporation method. A slide glass is cleaned in a piranha solution for 30 min at 80°C and then rinsed in deionized water and dried by nitrogen for 1 min. A UV-epoxy (NOA81, Thorlabs, Inc.) film with a thickness of 200 nm is prepared on the surface of the slide using a spin coater with speed of 3000 r/min and time of 50 s. The side of the slide glass with epoxy is close to the Ag-coated close-packed PS nanosphere mask, and then it is irradiated for 1 min under ultraviolet light (UVQ-702, LIENHE Fiber Optic Supplies, China) to promote the curing of the epoxy as shown in Fig. 2(b). Due to the PS nanospheres tightly attached to the surface of the slide glass, when the slide glass is removed from the mask, the PS nanospheres will be stripped simultaneously. Thus, the ATNA substrate is formatted and located on the surface of the silicon wafer as shown in Fig. 2(c).
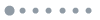
Figure 2.Fabrication and characterization of the ATNA substrates. (a)–(c) Sketch map of the fabrication process of the ATNA substrates; (d) SEM image of the Ag-coated PS nanosphere array with the diameter of PS nanospheres of ; (e) SEM image of the Ag-coated PS nanospheres stripped from the silicon wafer using the slide glass; SEM images of the ATNA substrates fabricated using PS nanospheres with (f) , (g) 400 nm, and (h) 600 nm. (i) Reflection spectra of the ATNA substrates with (red curve), 400 nm (green curve), and 600 nm (violet curve).
Figure 2(d) is the scanning electron microscope (SEM) image of the Ag-coated monolayer ordered hexagonally close-packed PS nanospheres array with diameter of PS nanospheres of . Figure 2(e) is the SEM image of the Ag-coated PS nanospheres stripped from the silicon wafer using the slide glass; note that the ordered PS microspheres are successfully stripped off by using the UV-epoxy film. Figure 2(f) is the corresponding SEM image of the ATNA substrate located on the silicon wafer. Note that the 2D ordering feature is well preserved in the resultant ATNA substrate. Figures 2(g) and 2(h) are the SEM images of the ATNA substrates obtained using the PS nanospheres with diameter of and 600 nm, respectively. It can be known that the interval and size of the ATNA substrates can be prepared controllably by adjusting the diameter of PS nanospheres. Figure 2(i) shows the measured reflection spectra of the ATNA substrates fabricated using PS nanospheres with , 400, and 600 nm, respectively. Note that the ATNA substrates fabricated with different sizes all have the LSPR effect in the visible band, and the resonance wavelength is blue-shifted with the increase of the diameter of the PS nanospheres.
B. Calculation of Electric-Field Enhancement
The focused characteristics of LPB and AVB are calculated with and the laser wavelength of . The transverse-electric-field intensity distributions of the focused LPB and AVB are shown in Figs. 3(a) and 3(b), respectively. It can be known that the focused LPB and AVB maintain the intensity and polarization characteristics. In addition, based on the horizontal intensity distribution through the centers of the focused LPB and AVB, as the green curves shown in Figs. 3(a) and 3(b), note that the transverse mode intensity size of the focused AVB is , which is larger than that of the focused LPB of .
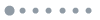
Figure 3.Calculation of the electric-field intensity enhancement factor of the ATNA substrates excited via the focused LPB and AVB. Transverse-electric-field intensity distributions of the focused (a) LPB and (b) AVB, under conditions of and . Sketch map of the ATNA substrates excited via (c) LPB and (d) AVB. Electric-field intensity distribution on the surface of ATNA substrates, with , excited via (e) LPB and (f) AVB. Electric-field intensity distribution on the surface of the ATNA substrates, with (g) and (h) 400 nm, excited via AVB.
Due to the periodic arrangement of the ATNA substrate, to simplify calculations, only one ATNA unit is adopted to calculate the electric-field intensity enhancement of the ATNA substrate excited via the focused LPB and AVB based on the 3D FDTD method. The ATNA unit is vertically illuminated via the focused LPB and AVB, as respectively shown in Figs. 3(c) and 3(d), in which the arrows represent the polarization direction of the two excitation beams. Note that the ATNA unit has radial symmetry [45,46]. The azimuthal polarization component of AVB is perpendicular to the tips of the ATNA unit, but the polarization component of the LPB can only be perpendicular to the tips of a part of the ATNA unit. Therefore, AVB excitation can achieve better electric-field enhancement characteristics on the tips of the ATNA unit than LPB excitation [52].
Figures 3(e) and 3(f) are the calculated electric-field intensity enhancements of the ATNA substrate with , illuminated via the focused LPB and AVB, respectively. The calculated results show that the ATNA substrate can achieve surface local mode generation at the apex of the triangular nanoprisms under excitation of two beams with different polarization and mode size. However, the electric-field intensity enhancement factor of , under excitation of the focused AVB, is larger than that of LPB excitation with . The main reason is that the mode size of the focused AVB matches well the geometric size of the nanoprism array unit, and thus the doughnut-like intensity distribution can effectively focus the excitation energy on the nanoprism array unit. Furthermore, the azimuthal polarization component of AVB is perpendicular to the tips of the nanoprism array unit compared with LPB, whose linear polarization component can only be perpendicular to the tips of a part of the ATNA unit. Figures 3(e) and 3(f) are the calculated electric-field intensity enhancements of the ATNA substrates fabricated using PS nanospheres with and 400 nm, respectively, under excitation of the focused AVB. Note that the EFs of the two ATNA substrates are smaller than that of the ATNA substrate with . The main reason is that the dimensions of the “unit cell” of the ATNA are mismatched with the dimensions and electric-field distribution of the AVB. In addition, it can also be seen that the EF of the ATNA substrate with is higher than that of the ATNA substrate with , because the geometric size of the ATNA substrate with is closer to the mode size of the excitation light compared with the ATNA substrate with .
C. SERS Performance of ATNA Substrates
1. Examination of SERS Sensitivity
Rhodamine 6G (R6G) is used as the probe molecule to characterize the SERS performance of the ATNA substrates. R6G is diluted to a specific concentration with anhydrous ethanol, and then the R6G solution (10 μL) is transferred to the ATNA substrate using a pipette. The anhydrous ethanol quickly evaporates, and then R6G molecules are left on the surface of the ATNA substrate. The power of the excitation light is set to 3.5 mW, and the integration time is 10 s. Figure 4(a) shows the Raman spectra of R6G, with concentrations of , and , absorbed on the surface of the ATNA substrates with and excited via the focused LPB. It can be seen that the SERS sensitivity of the ATNA substrate is with R6G molecules, because all the characteristic peaks of R6G can be distinguished clearly with a concentration of , yet they are unable to be excited with concentration down to . Figure 4(b) shows the Raman spectra of R6G with concentration of excited via the focused LPB and AVB, respectively. The examination result shows that the intensity of the Raman characteristic peaks excited via AVB (blue curve) is 3 times stronger than that of LPB excitation, revealing that the EF of the ATNA substrate excited via AVB is higher than that of LPB excitation. Figure 4(c) is the Raman spectra of R6G, with concentrations of and , excited via AVB. Note that all the characteristic peaks of R6G can be distinguished clearly with the concentration down to , in case of excitation via AVB, revealing that the SERS sensitivity is increased by 2 orders of magnitude compared to LPB excitation. It provides a possibility that the SERS sensitivity can still be increased by adjusting the polarization characteristics of the excitation light, even if the structure of the SERS substrate remains unchanged.
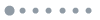
Figure 4.SERS sensitivity examination of the ATNA substrate with . (a) Raman spectra of R6G, with concentration from down to , absorbed on the surface of the ATNA substrates and excited via LPB. (b) Raman spectra of R6G, with concentration of , excited via AVB (blue curve) and LPB (red curve). (c) Raman spectra of R6G, with concentrations of and , excited via the focused AVB.
Keeping the power of excitation light and integration time unchanged, the SERS performance of the ATNA substrate with is also examined with R6G, under excitation of LPB and AVB. Figure 5(a) shows the Raman spectra of R6G, with concentrations of , , and , absorbed on the ATNA substrate with and excited using the LPB. Note that the SERS sensitivity of the ATNA substrate with is under excitation of the LPB. By changing the excitation source to AVB, the SERS sensitivity of the ATNA substrate with can be increased to as shown in Fig. 5(b). The SERS sensitivity is enhanced by selecting the AVB as the excitation source, but it is smaller than the SERS sensitivity () of the ATNA substrate with . In addition, this examination result also coincides with the theoretical calculation that the EF of the ATNA substrate with is smaller than that of the ATNA substrate with , due to the mode size of the focused AVB not matching well the structure size of the ATNA substrate with .
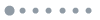
Figure 5.SERS sensitivity examination of the ATNA substrate with . (a) Raman spectra of R6G, with concentration from down to , absorbed on ATNA substrate and excited via LPB. (b) Raman spectra of R6G, with concentrations of , and , excited via AVB.
2. Examination of SERS Uniformity and Raman Enhancement Factor
To investigate the SERS uniformity of the ATNA substrate with under excitation of the focused AVB, Raman mapping is performed by using a home-built Raman mapping system as schematically shown in Fig. 1. A region with a size of , as shown in Fig. 6(a), is scanned with step value of 270 nm. To ensure the accuracy of Raman mapping, R6G solution of is absorbed on the surface of the ATNA substrate with , and the integration time for each point is set to 1 s. Figure 6(b) is the Raman mapping result reconstituted with the Raman characteristic peak of as shown in Fig. 6(b) inset. Furthermore, a line scan of Raman mapping is taken along the white curve in Fig. 6(b), as denoted by the histogram result in Fig. 6(c), with a deviation of less than from the average Raman signal intensity. The result exhibits that the ANTA substrate with retains high SERS uniformity under excitation of the focused AVB.
![Examination of SERS uniformity and Raman enhancement factor of the ATNA substrate with D=600 nm. (a) Schematic diagram of Raman mapping excited via AVB; (b) Raman imaging within a square of 15 μm×15 μm using the characteristic peak of 1511 cm−1 [inset in (d)] of R6G with a concentration of 10−8 M; (c) histogram of the intensities of the 1511 cm−1 characteristic peak obtained along the white curve in (b); (d) Raman spectra of R6G with concentrations of 10−8 M (red curve) and 10−1 M (black curve) on the ATNA substrate and a glass slide, respectively.](/Images/icon/loading.gif)
Figure 6.Examination of SERS uniformity and Raman enhancement factor of the ATNA substrate with . (a) Schematic diagram of Raman mapping excited via AVB; (b) Raman imaging within a square of using the characteristic peak of [inset in (d)] of R6G with a concentration of ; (c) histogram of the intensities of the characteristic peak obtained along the white curve in (b); (d) Raman spectra of R6G with concentrations of (red curve) and (black curve) on the ATNA substrate and a glass slide, respectively.
Under excitation of the focused AVB, the Raman enhancement factor of the ATNA substrate with is further estimated with the Raman spectrum of and of R6G solution absorbed on the ATNA substrate and a glass slide, respectively, as shown in Fig. 6(d). The intensity of the Raman characteristic peak of is selected to estimate the Raman enhancement factor, and the result is . Since the R6G molecules have random orientation over the surface of the ATNA substrate, the Raman enhancement factor is not affected by the Raman tensor of the molecules [53,54].
3. Examination of Reproducibility
To examine the reproducibility of the ATNA substrate, five ATNA substrates are prepared, and then R6G solutions with concentration of are absorbed on the five ATNA substrates. Under excitation of the focused AVB, the batch-to-batch Raman spectra examination result is shown in Fig. 7(a). The intensity variation of the characteristic peak of the five ATNA substrates is shown in Fig. 7(b), and the relative standard deviation (RSD) from the five ATNA substrates is calculated to be [13], revealing excellent reproducibility of the fabricated ATNA substrates.
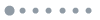
Figure 7.Examination of reproducibility of ATNA substrates excited via AVB. (a) Raman spectra of R6G with a concentration of obtained from five ANTA substrates with . (b) Histogram of intensities of the characteristic peak shown in (a).
4. CONCLUSION
In conclusion, a method for enhancing SERS performance of the ATNA substrate excited via the focused AVB has been presented. ATNA substrates with different structure sizes have been prepared based on the self-assembled and modified film lift-off method. Theoretical simulation shows that AVB excitation can achieve greater electric-field enhancement than LPB excitation. Furthermore, the experimental result proves that the SERS sensitivity obtained via the AVB excitation is using R6G, and it is 2 orders of magnitude lower than that of the LPB excitation of . The uniformity and reproducibility of the ATNA substrates are also respectively examined using Raman mapping and batch-to-batch measurement, and the Raman enhancement factor is estimated to be . This vector light field excitation method may provide a way to improve the SERS performance of the substrate in fields of highly sensitive Raman detection.